
биохимия атеросклероза
.pdf48Sereyrath Ngeth and Gordon A. Francis
4.Glomset JA: The plasma lecithin: cholesterol acyltransferase reaction. J Lipid Res
9:155–167, 1968.
5.Francis GA, Knopp RH, and Oram JF: Defective removal of cellular cholesterol and phospholipids by apolipoprotein A-I in Tangier disease. J Clin Invest 96: 78–87, 1995.
6.Walter M, Gerdes U, Seedorf U, and Assmann G: The high density lipoproteinand apolipoprotein A-I-induced mobilization of cellular cholesterol is impaired in fibroblasts from Tangier disease subjects. Biochem Biophys Res Commun
205:850–856, 1994.
7.Remaley AT, Schumacher UK, Stonik JA, Farsi BD, Nazih H, and Brewer HB Jr: Decreased reverse cholesterol transport from Tangier disease fibroblasts. Acceptor specificity and effect of brefeldin on lipid efflux. Arterioscler Thromb Vasc Biol 17: 1813–1821, 1997.
8.Bodzioch M, Orso E, Klucken J, Langmann T, Bottcher A, Diederich W, Drobnik W, Barlage S, Buchler C, Porsch-Ozcurumez M, Kaminski WE, Hahmann HW, Oette K, Rothe G, Aslanidis C, Lackner KJ, and Schmitz G: The gene encoding ATP-binding cassette transporter 1 is mutated in Tangier disease. Nat Genet 22: 347–351, 1999.
9.Brooks-Wilson A, Marcil M, Clee SM, Zhang LH, Roomp K, van Dam M, Yu L, Brewer C, Collins JA, Molhuizen HO, Loubser O, Ouelette BF, Fichter K, Ashbourne-Excoffon KJ, Sensen CW, Scherer S, Mott S, Denis M, Martindale D, Frohlich J, Morgan K, Koop B, Pimstone S, Kastelein JJ, and Hayden MR: Mutations in ABC1 in Tangier disease and familial high-density lipoprotein deficiency. Nat Genet 22: 336–345, 1999.
10.Rust S, Rosier M, Funke H, Real J, Amoura Z, Piette JC, Deleuze JF, Brewer HB, Duverger N, Denefle P, and Assmann G: Tangier disease is caused by mutations in the gene encoding ATP-binding cassette transporter 1. Nat Genet 22: 352–355, 1999.
11.Lawn RM, Wade DP, Garvin MR, Wang X, Schwartz K, Porter JG, Seilhamer JJ, Vaughan AM, and Oram JF: The Tangier disease gene product ABC1 controls the cellular apolipoprotein-mediated lipid removal pathway. J Clin Invest 104: R25–R31, 1999.
12.Brousseau ME, Schaefer EJ, Dupuis J, Eustace B, Van Eerdewegh P, Goldkamp AL, Thurston LM, FitzGerald MG, Yasek-McKenna D, O’Neill G, Eberhart GP, Weiffenbach B, Ordovas JM, Freeman MW, Brown RH Jr, and Gu JZ: Novel mutations in the gene encoding ATP-binding cassette 1 in four tangier disease kindreds. J Lipid Res 41: 433–441, 2000.
13.Dean M, Hamon Y, and Chimini G: The human ATP-binding cassette (ABC) transporter superfamily. J Lipid Res 42: 1007–1017, 2001.
14.Oram JF: ATP-binding cassette transporter A1 and cholesterol trafficking. Curr Opin Lipidol 13: 373–381, 2002.
15.Assmann G, Von Eckardstein A, and Brewer HB: Familial Analphalipoproteinemia: Tangier Disease. In: Scriver CR, Beaudet AL, Sly WS, and Valle D (eds), The Metabolic and Molecular Bases of Inherited Disease, 8th edn. McGraw-Hill, New York, 2001, pp. 2937–2980.
16.Asztalos BF, Brousseau ME, McNamara JR, Horvath KV, Roheim PS, and Schaefer EJ: Subpopulations of high density lipoproteins in homozygous and heterozygous Tangier disease. Atherosclerosis 156: 217–225, 2001.
Chapter 3. Howdy Partner: Apolipoprotein A-I–ABCA1 Interactions |
49 |
17.Serfaty-Lacrosniere C, Civeira F, Lanzberg A, Isaia P, Berg J, Janus ED, Smith MP, Pritchard PH, Frohlich J, Lees RS, Barnard GF, Ordovas JM, and Schaefer EJ: Homozygous Tangier disease and cardiovascular disease. Atherosclerosis 107: 85–98, 1994.
18.Clee SM, Kastelein JJ, van Dam M, Marcil M, Roomp K, Zwarts KY, Collins JA, Roelants R, Tamasawa N, Stulc T, Suda T, Ceska R, Boucher B, Rondeau C, DeSouich C, Brooks-Wilson A, Molhuizen HO, Frohlich J, Genest J Jr, and Hayden MR: Age and residual cholesterol efflux affect HDL cholesterol levels and coronary artery disease in ABCA1 heterozygotes. J Clin Invest 106: 1263–1270, 2000.
19.Langmann T, Klucken J, Reil M, Liebisch G, Luciani MF, Chimini G, Kaminski WE, and Schmitz G: Molecular cloning of the human ATP-binding cassette transporter 1 (hABC1): evidence for sterol-dependent regulation in macrophages. Biochem Biophys Res Commun 257: 29–33, 1999.
20.Costet P, Luo Y, Wang N, and Tall AR: Sterol-dependent transactivation of the ABC1 promoter by the liver X receptor/retinoid X receptor. J Biol Chem 275: 28240–28245, 2000.
21.Schwartz K, Lawn RM, and Wade DP: ABC1 gene expression and ApoA-I-medi- ated cholesterol efflux are regulated by LXR. Biochem Biophys Res Commun
274:794–802, 2000.
22.Francis GA, Annicotte JS, and Auwerx J: Liver X receptors: Xcreting Xol to combat atherosclerosis. Trends Mol Med 8: 455–458, 2002.
23.Bortnick AE, Rothblat GH, Stoudt G, Hoppe KL, Royer LJ, McNeish J, and Francone OL: The correlation of ATP-binding cassette 1 mRNA levels with cholesterol efflux from various cell lines. J Biol Chem 275: 28634–28640, 2000.
24.Remaley AT, Stonik JA, Demosky SJ, Neufeld EB, Bocharov AV, Vishnyakova TG, Eggerman TL, Patterson AP, Duverger NJ, Santamarina-Fojo S, and Brewer HB Jr: Apolipoprotein specificity for lipid efflux by the human ABCAI transporter. Biochem Biophys Res Commun 280: 818–823, 2001.
25.Oram JF, Lawn RM, Garvin MR, and Wade DP: ABCA1 is the cAMP-inducible apolipoprotein receptor that mediates cholesterol secretion from macrophages. J Biol Chem 275: 34508–34511, 2000.
26.Wang N, Silver DL, Thiele C, and Tall AR: ATP-binding cassette transporter A1 (ABCA1) functions as a cholesterol efflux regulatory protein. J Biol Chem
276:23742–23747, 2001.
27.Frank PG and Marcel YL: Apolipoprotein A-I: structure–function relationships. J Lipid Res 41: 853–872, 2000.
28.Oram JF: HDL apolipoproteins and ABCA1: partners in the removal of excess cellular cholesterol. Arterioscler Thromb Vasc Biol 23: 720–727, 2003.
29.Hamon Y, Broccardo C, Chambenoit O, Luciani MF, Toti F, Chaslin S, Freyssinet JM, Devaux PF, McNeish J, Marguet D, and Chimini G: ABC1 promotes engulfment of apoptotic cells and transbilayer redistribution of phosphatidylserine. Nat Cell Biol 2: 399–406, 2000.
30.Lin G and Oram JF: Apolipoprotein binding to protruding membrane domains during removal of excess cellular cholesterol. Atherosclerosis 149: 359–370, 2000.
31.Wang N, Silver DL, Costet P, and Tall AR: Specific binding of ApoA-I, enhanced cholesterol efflux, and altered plasma membrane morphology in cells expressing ABC1. J Biol Chem 275: 33053–33058, 2000.
50 Sereyrath Ngeth and Gordon A. Francis
32.Vaughan AM and Oram JF: ABCA1 redistributes membrane cholesterol independent of apolipoprotein interactions. J Lipid Res 44: 1373–1380, 2003.
33.Gillotte KL, Davidson WS, Lund-Katz S, Rothblat GH, and Phillips MC: Removal of cellular cholesterol by pre-beta-HDL involves plasma membrane microsolubilization. J Lipid Res 39: 1918–1928, 1998.
34.Gillotte KL, Zaiou M, Lund-Katz S, Anantharamaiah GM, Holvoet P, Dhoest A, Palgunachari MN, Segrest JP, Weisgraber KH, Rothblat GH, and Phillips MC: Apolipoprotein-mediated plasma membrane microsolubilization. J Biol Chem 274: 2021–2028, 1999.
35.Chambenoit O, Hamon Y, Marguet D, Rigneault H, Rosseneu M, and Chimini G: Specific docking of apolipoprotein A-I at the cell surface requires a functional ABCA1 transporter. J Biol Chem 276: 9955–9960, 2001.
36.Rigot V, Hamon Y, Chambenoit O, Alibert M, Duverger N, and Chimini G: Distinct sites on ABCA1 control distinct steps required for cellular release of phospholipids. J Lipid Res 43: 2077–2086, 2002.
37.Smith JD, Waelde C, Horwitz A, and Zheng P: Evaluation of the role of phosphatidylserine translocase activity in ABCA1-mediated lipid efflux. J Biol Chem 277: 17797–17803, 2002.
38.Denis M, Haidar B, Marcil M, Bouvier M, Krimbou L, and Genest J Jr: Molecular and cellular physiology of apolipoprotein A-I lipidation by the ATPbinding cassette transporter A1 (ABCA1). J Biol Chem 279: 7384–7394, 2004.
39.Fitzgerald ML, Morris AL, Chroni A, Mendez AJ, Zannis VI, and Freeman MW: ABCA1 and amphipathic apolipoproteins form high-affinity molecular complexes required for cholesterol efflux. J Lipid Res 45: 287–294, 2004.
40.Fitzgerald ML, Morris AL, Rhee JS, Andersson LP, Mendez AJ, and Freeman MW: Naturally occurring mutations in the largest extracellular loops of ABCA1 can disrupt its direct interaction with apolipoprotein A-I. J Biol Chem 277: 33178–33187, 2002.
41.Chroni A, Liu T, Fitzgerald ML, Freeman MW, and Zannis VI: Cross-linking and lipid efflux properties of apoA-I mutants suggest direct association between apoA-I helices and ABCA1. Biochemistry 43: 2126–2139, 2004.
42.Denis M, Haidar B, Marcil M, Bouvier M, Krimbou L, and Genest J: Characterization of oligomeric human ATP binding cassette transporter A1. Potential implications for determining the structure of nascent high density lipoprotein particles. J Biol Chem 279: 41529–41536, 2004.
43.Panagotopulos SE, Witting SR, Horace EM, Hui DY, Maiorano JN, and Davidson WS: The role of apolipoprotein A-I helix 10 in apolipoproteinmediated cholesterol efflux via the ATP-binding cassette transporter ABCA1. J Biol Chem 277: 39477–39484, 2002.
44.Neufeld EB, Remaley AT, Demosky SJ, Stonik JA, Cooney AM, Comly M, Dwyer NK, Zhang M, Blanchette-Mackie J, Santamarina-Fojo S, and Brewer HB Jr: Cellular localization and trafficking of the human ABCA1 transporter. J Biol Chem 276: 27584–27590, 2001.
45.Orso E, Broccardo C, Kaminski WE, Bottcher A, Liebisch G, Drobnik W, Gotz A, Chambenoit O, Diederich W, Langmann T, Spruss T, Luciani MF, Rothe G, Lackner KJ, Chimini G, and Schmitz G: Transport of lipids from Golgi to plasma membrane is defective in Tangier disease patients and Abc1-deficient mice. Nat Genet 24: 192–196, 2000.
Chapter 3. Howdy Partner: Apolipoprotein A-I–ABCA1 Interactions |
51 |
46.Chen W, Sun Y, Welch C, Gorelik A, Leventhal AR, Tabas I, and Tall AR: Preferential ATP-binding cassette transporter A1-mediated cholesterol efflux from late endosomes/lysosomes. J Biol Chem 276: 43564–43569, 2001.
47.Yamauchi Y, Chang CC, Hayashi M, Abe-Dohmae S, Reid PC, Chang TY, and Yokoyama S: Intracellular cholesterol mobilization involved in the ABCA1/
apolipoprotein-mediated assembly of high density lipoprotein in fibroblasts. J Lipid Res 45: 1943–1951, 2004.
48.Zha X, Gauthier A, Genest J, and McPherson R: Secretory vesicular transport from the Golgi is altered during ATP-binding cassette protein A1 (ABCA1)- mediated cholesterol efflux. J Biol Chem 278: 10002–10005, 2003.
49.Takahashi Y and Smith JD: Cholesterol efflux to apolipoprotein AI involves endocytosis and resecretion in a calcium-dependent pathway. Proc Natl Acad Sci U S A 96: 11358–11363, 1999.
50.Neufeld EB, Stonik JA, Demosky SJ Jr, Knapper CL, Combs CA, Cooney A, Comly M, Dwyer N, Blanchette-Mackie J, Remaley AT, Santamarina-Fojo S, and Brewer HB Jr: The ABCA1 transporter modulates late endocytic trafficking: insights from the correction of the genetic defect in Tangier disease. J Biol Chem 279: 15571–15578, 2004.
51.Tanaka AR, Abe-Dohmae S, Ohnishi T, Aoki R, Morinaga G, Okuhira K, Ikeda Y, Kano F, Matsuo M, Kioka N, Amachi T, Murata M, Yokoyama S, and Ueda K: Effects of mutations of ABCA1 in the first extracellular domain on subcellular trafficking and ATP binding/hydrolysis. J Biol Chem 278: 8815–8819, 2003.
52.Albrecht C, Baynes K, Sardini A, Schepelmann S, Eden ER, Davies SW, Higgins CF, Feher MD, Owen JS, and Soutar AK: Two novel missense mutations in ABCA1 result in altered trafficking and cause severe autosomal recessive HDL deficiency. Biochim Biophys Acta 1689: 47–57, 2004.
53.Schmitz G, Kaminski WE, and Orso E: ABC transporters in cellular lipid trafficking. Curr Opin Lipidol 11: 493–501, 2000.
54.Krimbou L, Denis M, Haidar B, Carrier M, Marcil M, and Genest J Jr: Molecular interactions between apoE and ABCA1: impact on apoE lipidation. J Lipid Res 45: 839–848, 2004.
55.Williams DL, de La Llera-Moya M, Thuahnai ST, Lund-Katz S, Connelly MA, Azhar S, Anantharamaiah GM, and Phillips MC: Binding and cross-linking studies show that scavenger receptor BI interacts with multiple sites in apolipoprotein A-I and identify the class A amphipathic alpha-helix as a recognition motif. J Biol Chem 275: 18897–18904, 2000.
56.Fitzgerald ML, Okuhira K, Short GF III, Manning JJ, Bell SA, and Freeman MW: ATP-binding cassette transporter A1 contains a novel C-terminal VFVNFA motif that is required for its cholesterol efflux and ApoA-I binding activities. J Biol Chem 279: 48477–48485, 2004.
57.See RH, Caday-Malcolm RA, Singaraja RR, Zhou S, Silverston A, Huber MT, Moran J, James ER, Janoo R, Savill JM, Rigot V, Zhang LH, Wang M, Chimini G, Wellington CL, Tafuri SR, and Hayden MR: Protein kinase A site-specific phosphorylation regulates ATP-binding cassette A1 (ABCA1)-mediated phospholipid efflux. J Biol Chem 277: 41835–41842, 2002.
58.Haidar B, Denis M, Krimbou L, Marcil M, and Genest J Jr: cAMP induces ABCA1 phosphorylation activity and promotes cholesterol efflux from fibroblasts. J Lipid Res 43: 2087–2094, 2002.
52 Sereyrath Ngeth and Gordon A. Francis
59.Haidar B, Denis M, Marcil M, Krimbou L, and Genest J Jr: Apolipoprotein A-I activates cellular cAMP signaling through the ABCA1 transporter. J Biol Chem 279: 9963–9969, 2004.
60.Mendez AJ, Oram JF, and Bierman EL: Protein kinase C as a mediator of high density lipoprotein receptor-dependent efflux of intracellular cholesterol. J Biol Chem 266: 10104–10111, 1991.
61.Li Q and Yokoyama S: Independent regulation of cholesterol incorporation into free apolipoprotein-mediated cellular lipid efflux in rat vascular smooth muscles cells. J Biol Chem 270: 26216–26223, 1995.
62.Li Q, Tsujita M, and Yokoyama S: Selective down-regulation by protein kinase C inhibitors of apolipoprotein-mediated cellular cholesterol efflux in macrophages. Biochemistry 36: 12045–12052, 1997.
63.Yamauchi Y, Hayashi M, Abe-Dohmae S, and Yokoyama S: Apolipoprotein A-I activates protein kinase C alpha signaling to phosphorylate and stabilize ATP binding cassette transporter A1 for the high density lipoprotein assembly. J Biol Chem 278: 47890–47897, 2003.
64.Tang C, Vaughan AM, and Oram JF: Janus kinase 2 modulates the apolipoprotein interactions with ABCA1 required for removing cellular cholesterol. J Biol Chem 279: 7622–7628, 2004.
65.Roosbeek S, Peelman F, Verhee A, Labeur C, Caster H, Lensink MF, Cirulli C, Grooten J, Cochet C, Vandekerckhove J, Amoresano A, Chimini G, Tavernier J, and Rosseneu M: Phosphorylation by protein kinase CK2 modulates the activity of the ATP binding cassette A1 transporter. J Biol Chem 279: 37779–37788, 2004.
66.Arakawa R and Yokoyama S: Helical apolipoproteins stabilize ATP-binding cassette transporter A1 by protecting it from thiol protease-mediated degradation. J Biol Chem 277: 22426–22429, 2002.
67.Wang N, Chen W, Linsel-Nitschke P, Martinez LO, Agerholm-Larsen B, Silver DL, and Tall AR: A PEST sequence in ABCA1 regulates degradation by calpain protease and stabilization of ABCA1 by apoA-I. J Clin Invest 111: 99–107, 2003.
68.Martinez LO, Agerholm-Larsen B, Wang N, Chen W, and Tall AR: Phosphorylation of a pest sequence in ABCA1 promotes calpain degradation and is reversed by ApoA-I. J Biol Chem 278: 37368–37374, 2003.
69.Chen W, Wang N, and Tall AR: A PEST deletion mutant of ABCA1 shows impaired internalization and defective cholesterol efflux from late endosomes. J Biol Chem 280: 29277–29281, 2005.
Biochemistry of Atherosclerosis edited by S.K. Cheema, Springer, New York, 2006
4
Role of the Scavenger Receptor Class B Type I in Lipoprotein Metabolism and Atherosclerosis: Insights from Genetically Altered Mice
BERNARDO TRIGATTI
Abstract
The scavenger receptor class B type I (SR-BI) is a cell surface multiligand receptor that can bind high-density lipoproteins and mediate exchange of lipids with cells. It is expressed abundantly in liver and in cells of the vascular wall. Analyses of genetically manipulated mice with altered levels of SR-BI expression in different tissues have yielded important insight into its roles in HDL metabolism and atherosclerosis.
Keywords: atherosclerosis; eNOS; HDL; knockout; liver; macrophage; PDZK1; SR-BI; transgenic
Abbreviations: ABC, ATP-binding cassette transporter; AGE-BSA, advance glycation end-product modified bovine serum albumin; CETP, cholesteryl ester transfer protein; eNOS, endothelial nitric oxide synthase; HDL, high-density lipoprotein; KO, knockout; LCAT, lecithin cholesterol acyltransferase; LDL, low-density lipoprotein; MDCK, Madin–Darby canine kidney; PAF-AH, platelet activating factor acylhydrolase; PDZ, Postsynaptic density protein-95/Drosophila discs-large/ZO1; PKA, protein kinase A; PL, phospholipid; PON, paraoxonase; SPAP, small PDZK1-associated protein; SAA, serum amyloid alpha; SR-BI, scavenger receptor class B type I; VLDL, very low-density lipoprotein
Introduction
Clinical, epidemiological, and basic research points to a role for high-density lipoproteins (HDL) in protection against atherosclerosis and heart disease [1–3]. Because of this relationship, treatments aimed at increasing HDL function have garnered great interest [3]. Efforts over the past several years have been aimed at attempting to understand the molecular and physiological mechanisms by which HDL protects against atherosclerosis. A variety of
53
54 Bernardo Trigatti
pathways appear to be involved. For example, HDL is a carrier of antioxidant vitamins (such as α-tocopherol—vitamin E), which help protect cells from harmful effects of reactive oxygen species [4, 5]. HDL is also associated with enzymes such as paraoxonase (PON) and platelet activating factor acylhydrolase (PAF-AH) that may play important roles in the removal of oxidized lipids from other lipoproteins such as low-density lipoprotein (LDL) [6–8]. HDLs also trigger signaling events within cells leading to diverse consequences, including the activation of endothelial nitric oxide synthase (eNOS) and protection of cells in the vascular wall against apoptosis, processes which have been associated with protection against atherosclerosis [9–18].
HDL also plays a key role in reverse cholesterol transport. This is the removal of cholesterol from cells in peripheral tissues (e.g., macrophages and foam cells in the artery wall), and its delivery to the liver. HDL-associated cholesterol is converted to cholesteryl ester by lecithin cholesterol acyltransferase (LCAT) [19, 20]. HDL-associated cholesteryl ester can be transferred to very low-density lipoprotein (VLDL) in exchange for triglycerides by cholesteryl ester transfer protein (CETP) [21]. Alternatively, cholesteryl ester that remains associated with HDL can be taken up by liver hepatocytes via a pathway called selective lipid uptake. This involves the net internalization of only the lipid portion of the HDL particle, without the net internalization and degradation of the HDL particle itself (reviewed in [22]). Internalized cholesterol can be secreted into bile either as cholesterol or after conversion to bile acids or be repackaged into nascent VLDL and secreted from the hepatocyte into the circulation (reviewed in [22]). HDL has also been implicated in the reverse transport of oxidized lipids, derived from cells or from other lipoproteins, to the liver for clearance [23]. Therefore, the “reverse cholesterol transport” pathway may play important roles in the clearance of diverse lipids in addition to cholesterol, highlighting the importance of this pathway to protection against atherosclerosis.
The Scavenger Receptor Class B Type I
Almost a decade ago, Krieger and coworkers [24] identified the scavenger receptor class B type I (SR-BI) as an HDL receptor that could mediate the selective uptake of HDL lipids. SR-BI is a member of the CD36 family of proteins (see Chapter 5) and contains two transmembrane domains, short N-terminal and C-terminal cytoplasmic regions, and a large extracellular region that is heavily glycosylated (reviewed in [22], see Fig. 4.1). SR-BI is also palmitoylated on two Cys residues near the junction between the C-terminal cytoplasmic and transmembrane regions [25, 26]. It is expressed most abundantly in cells with a high capacity for selective HDL lipid uptake—steroidogenic cells and hepatocytes (reviewed in [22]). This suggested that SR-BI may play an important role in the later stages of reverse
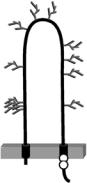
Chapter 4. Role of the Scavenger Receptor-BI and Atherosclerosis |
55 |
||
HDL |
Acetyl-LDL |
Anionic PL |
|
LDL |
Oxidized LDL |
Apoptotic Cells |
|
VLDL |
Oxidized HDL |
LPS |
|
Chylomicrons |
AGE-BSA |
SAA |
|
Apolipoproteins |
|
|
|
SR-BI
c c
N
c
FIGURE 4.1. SR-BI and known ligands. SR-BI is represented with two transmembrane regions residing in the membrane (gray bar), cytoplasmic C-terminus and N-terminus, and a large extracellular region. Approximate locations of N-linked glycosylation sites are shown in gray, as are the locations of two cysteine residues that are sites of palmitoylation. Known SR-BI ligands are listed at the top and include lipoproteins, chemically modified proteins, and lipoproteins including advance glycation endproduct modified BSA (AGE-BSA), liposomes containing anionic phospholipids (PL), apoptotic cells, bacterial lipopolysaccharide, and the acute phase protein serum amyloid alpha (SAA) (either free or associated with lipoproteins) (reviewed in [27] and [86]).
cholesterol transport and transport of HDL cholesterol to steroidogenic tissues. SR-BI is also expressed in a variety of other cell types (reviewed in [22, 27, 28]) including macrophages and endothelial cells [29–32] and could therefore influence atherosclerosis locally at the vessel wall (explained later).
When overexpressed in transfected cells, SR-BI can mediate both increased selective uptake of HDL lipids and increased efflux of free cholesterol tracer to HDL acceptors [24, 33, 34]. This has led to the notion that SR-BI can mediate the bidirectional flux of lipids between cells and bound lipoproteins and the suggestion that the direction of net flux is dependent on the concentration gradient of the lipid [35]. For example, in Chinese hamster ovaryderived cells, which have relatively low levels of intracellular cholesterol, the net flux appears to be in the direction of the cell [24]. The observation that SR-BI can mediate the efflux of unesterified cholesterol tracer from cells has led to the suggestion that it may play an important role in HDL-dependent
56 Bernardo Trigatti
cholesterol efflux from macrophages (see [33, 34]). Consequently, SR-BI has been implicated in both early steps (i.e., cholesterol efflux from macrophages) and late steps (hepatic selective HDL cholesterol uptake) in reverse cholesterol transport.
SR-BI has also been implicated in mediating HDL-dependent signaling events in endothelial cells (see Chapter 5). For example, the HDL-dependent stimulation of eNOS appears to be dependent on SR-BI [9, 11, 12, 14]. This may occur via the transfer of bioactive lipids (estradiol, ceramide, and/or sphingosine-1-phosphate) with signaling activity and/or through the generation of intracellular signaling events including the activation PKB/Akt pathways [9, 11, 12, 14, 17]. The ability of SR-BI to mediate HDL-dependent eNOS activation may also be related to SR-BI-mediated cholesterol efflux to HDL [36]. The role of SR-BI in cholesterol efflux from cells appears to be controversial, however. Overexpression of SR-BI in transfected cells has been shown quite clearly to increase the efflux of cholesterol tracer to HDL [33, 34, 37]. In contrast, several studies have indicated that SR-BI is not required for cholesterol efflux to HDL in different cells including endothelial cells [38] and macrophages [39–41].
Certain tissues, including the liver, contain an alternatively spliced transcript giving rise to an isoform called SR-BII, which differs from SR-BI in its C-terminal cytoplasmic region [42, 43]. SR-BI appears to be considerably more active than SR-BII in mediating lipid transfer between cells and lipoproteins [44]. This may be the result of a different subcellular distribution of the two isoforms, with SR-BI demonstrating a greater distribution on the plasma membrane than does SR-BII [44].
Recently, Smart and coworkers [18] have reported that SR-BI expression induces apoptosis in cells in the absence of ligand stimulation. Both HDL interaction with SR-BI and eNOS activation were able to inhibit SR-BI-triggered apoptosis. This suggests the possibility that SR-BI could contribute to apoptosis of endothelial cells or other cells in an HDLand eNOS-regulated manner. This process could contribute to endothelial dysfunction and initiation or development of atherosclerosis, and HDL and eNOS might act, at least in part, by halting this process [18].
In addition to mediating the binding of HDL and HDL-dependent lipid exchange with cells, SR-BI also binds a variety of other ligands (Fig. 4.1). These include other lipoproteins (LDL, VLDL, and chylomicrons), modified proteins/lipoproteins (e.g., advance glycation end-product modified bovine serum albumin (AGE-BSA), oxidized or acetylated LDL), the acute phase protein serum amyloid alpha (SAA—either free or bound to HDL), phospholipid (PL) vesicles containing anionic PL, apoptotic cells, and bacterial cell surface components (e.g., lipopolysaccharide) (see [27] for recent review). Therefore in addition to playing a role in HDL metabolism, SR-BI may play roles in a variety of other physiological processes, many of which may affect the development or progression of atherosclerosis.
Chapter 4. Role of the Scavenger Receptor-BI and Atherosclerosis |
57 |
SR-BI and Atherosclerosis: Studies of Gene-Targeted
Mice
Studies of genetically manipulated mice have provided substantial insight into SR-BI’s physiological role in HDL metabolism and atherosclerosis. SR-BI knockout (KO) mice, in which the gene encoding SR-BI (Scarb1) has been inactivated by targeted mutagenesis, exhibit no detectable SR-BI expression in tissues examined [45]. These mice have increased cholesterol levels associated with enlarged HDL particles with altered compositions [45–48]. The enlarged HDL particles contain increased apoE and nonesterified/esterified cholesterol ratios accompanied by decreased levels of LCAT activity [45, 47–49]. These alterations in plasma HDL composition and structure are accompanied by reduced lipid storage in steroidogenic tissues and reduced biliary cholesterol secretion [45, 46, 50]. Mice that are heterozygous for the targeted mutation express half of normal SR-BI levels in tissues that have been analyzed, including liver and adrenal glands [45]. These mice have slightly increased HDL cholesterol, and ~50% reductions in adrenal cholesterol levels [45]. SR-BI KO mice do not, however, exhibit altered hepatic cholesterol levels or cholesterol biosynthesis [49, 50]. SR-BI KO mice have been reported to exhibit increased levels of hepatic expression of ABCG1, an ATP-binding cassette transporter (ABC) implicated in cholesterol efflux to HDL, and mice carrying one or two copies of the inactivating mutation exhibit reduced hepatic ABCG5 and ABCG8, other ABC family members which have been implicated in regulating hepatic cholesterol secretion into bile [49, 51, 52].
SR-BI KO mice have reduced hepatic and adrenal uptake of HDL cholesterol and exhibit reduced selective clearance of HDL and LDL cholesterol from plasma [53–55]. These studies suggest that SR-BI is required for selective uptake of cholesterol from HDL and LDL by liver and steroidogenic cells in vivo [45, 46, 50, 53–56]. SR-BI KO mice have also been reported to exhibit a greater increase in plasma triglycerides after an intragastric fat load whereas adenovirus-infected mice with hepatic overexpression of SR-BI show a reduced response, relative to controls [57, 58]. This combined with in vitro studies, demonstrating reduced binding of chylomicron-like particles to hepatocytes from SR-BI KO mice relative to controls, suggest that SR-BI may also be involved in chylomicron metabolism in vivo [57, 58].
SR-BI KO mice develop increased atherosclerosis either when challenged with high-fat/atherogenic diets or when SR-BI deficiency is combined with deficiency in genes encoding either LDL receptor or apoE [39, 46, 49]. Mice deficient in SR-BI alone develop increased diet-induced atherosclerosis compared to either heterozygous or homozygous SR-BI-expressing mice [49]. Examination of gene expression in aortas from these mice has revealed increased expression of genes involved in monocyte adhesion to endothelial cells, including P- and E-selectins and vascular cell adhesion molecule (VCAM)-1; similar increases were seen in CD68, apoE, ABCA1, and ABCG1,