
- •Preface
- •Contents
- •1 Nonideal plasma. Basic concepts
- •1.1 Interparticle interactions. Criteria of nonideality
- •1.1.1 Interparticle interactions
- •1.1.2 Coulomb interaction. Nonideality parameter
- •1.1.4 Compound particles in plasma
- •1.2.2 Metal plasma
- •1.2.3 Plasma of hydrogen and inert gases
- •1.2.4 Plasma with multiply charged ions
- •1.2.5 Dusty plasmas
- •1.2.6 Nonneutral plasmas
- •References
- •2.1 Plasma heating in furnaces
- •2.1.1 Measurement of electrical conductivity and thermoelectromotive force
- •2.1.2 Optical absorption measurements.
- •2.1.3 Density measurements.
- •2.1.4 Sound velocity measurements
- •2.2 Isobaric Joule heating
- •2.2.1 Isobaric heating in a capillary
- •2.2.2 Exploding wire method
- •2.3 High–pressure electric discharges
- •References
- •3.1 The principles of dynamic generation and diagnostics of plasma
- •3.2 Dynamic compression of the cesium plasma
- •3.3 Compression of inert gases by powerful shock waves
- •3.4 Isentropic expansion of shock–compressed metals
- •3.5 Generation of superdense plasma in shock waves
- •References
- •4 Ionization equilibrium and thermodynamic properties of weakly ionized plasmas
- •4.1 Partly ionized plasma
- •4.2 Anomalous properties of a metal plasma
- •4.2.1 Physical properties of metal plasma
- •4.2.2 Lowering of the ionization potential
- •4.2.3 Charged clusters
- •4.2.4 Thermodynamics of multiparticle clusters
- •4.3 Lowering of ionization potential and cluster ions in weakly nonideal plasmas
- •4.3.1 Interaction between charged particles and neutrals
- •4.3.2 Molecular and cluster ions
- •4.3.3 Ionization equilibrium in alkali metal plasma
- •4.4 Droplet model of nonideal plasma of metal vapors. Anomalously high electrical conductivity
- •4.4.1 Droplet model of nonideal plasma
- •4.4.2 Ionization equilibrium
- •4.4.3 Calculation of the plasma composition
- •4.5 Metallization of plasma
- •4.5.3 Phase transition in metals
- •References
- •5.1.1 Monte Carlo method
- •5.1.2 Results of calculation
- •5.1.4 Wigner crystallization
- •5.1.5 Integral equations
- •5.1.6 Polarization of compensating background
- •5.1.7 Charge density waves
- •5.1.8 Sum rules
- •5.1.9 Asymptotic expressions
- •5.1.10 OCP ion mixture
- •5.2 Multicomponent plasma. Results of the perturbation theory
- •5.3 Pseudopotential models. Monte Carlo calculations
- •5.3.1 Choice of pseudopotential
- •5.5 Quasiclassical approximation
- •5.6 Density functional method
- •5.7 Quantum Monte Carlo method
- •5.8 Comparison with experiments
- •5.9 On phase transitions in nonideal plasmas
- •References
- •6.1 Electrical conductivity of ideal partially ionized plasma
- •6.1.1 Electrical conductivity of weakly ionized plasma
- •6.2 Electrical conductivity of weakly nonideal plasma
- •6.3 Electrical conductivity of nonideal weakly ionized plasma
- •6.3.1 The density of electron states
- •6.3.2 Electron mobility and electrical conductivity
- •References
- •7 Electrical conductivity of fully ionized plasma
- •7.1 Kinetic equations and the results of asymptotic theories
- •7.2 Electrical conductivity measurement results
- •References
- •8 The optical properties of dense plasma
- •8.1 Optical properties
- •8.2 Basic radiation processes in rarefied atomic plasma
- •8.5 The principle of spectroscopic stability
- •8.6 Continuous spectra of strongly nonideal plasma
- •References
- •9 Metallization of nonideal plasmas
- •9.1 Multiple shock wave compression of condensed dielectrics
- •9.1.1 Planar geometry
- •9.1.2 Cylindrical geometry
- •9.3 Metallization of dielectrics
- •9.3.1 Hydrogen
- •9.3.2 Inert gases
- •9.3.3 Oxygen
- •9.3.4 Sulfur
- •9.3.5 Fullerene
- •9.3.6 Water
- •9.3.7 Dielectrization of metals
- •9.4 Ionization by pressure
- •References
- •10 Nonneutral plasmas
- •10.1.1 Electrons on a surface of liquid He
- •10.1.2 Penning trap
- •10.1.3 Linear Paul trap
- •10.1.4 Storage ring
- •10.2 Strong coupling and Wigner crystallization
- •10.3 Melting of mesoscopic crystals
- •10.4 Coulomb clusters
- •References
- •11 Dusty plasmas
- •11.1 Introduction
- •11.2 Elementary processes in dusty plasmas
- •11.2.1 Charging of dust particles in plasmas (theory)
- •11.2.2 Electrostatic potential around a dust particle
- •11.2.3 Main forces acting on dust particles in plasmas
- •11.2.4 Interaction between dust particles in plasmas
- •11.2.5 Experimental determination of the interaction potential
- •11.2.6 Formation and growth of dust particles
- •11.3 Strongly coupled dusty plasmas and phase transitions
- •11.3.1 Theoretical approaches
- •11.3.2 Experimental investigation of phase transitions in dusty plasmas
- •11.3.3 Dust clusters in plasmas
- •11.4 Oscillations, waves, and instabilities in dusty plasmas
- •11.4.1 Oscillations of individual particles in a sheath region of gas discharges
- •11.4.2 Linear waves and instabilities in weakly coupled dusty plasmas
- •11.4.3 Waves in strongly coupled dusty plasmas
- •11.4.4 Experimental investigation of wave phenomena in dusty plasmas
- •11.5 New directions in experimental research
- •11.5.1 Investigations of dusty plasmas under microgravity conditions
- •11.5.2 External perturbations
- •11.5.3 Dusty plasma of strongly asymmetric particles
- •11.5.4 Dusty plasma at cryogenic temperatures
- •11.5.5 Possible applications of dusty plasmas
- •11.6 Conclusions
- •References
- •Index
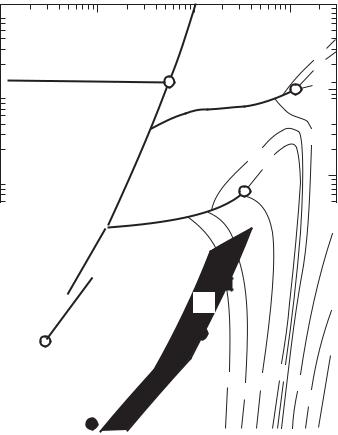
358 |
METALLIZATION OF NONIDEAL PLASMAS |
p, GPa
105
Solid metal
104
Tr4
Solid isolator
103
Tr3
102
He
Tr2 101
100
102 |
103 |
He++
1.99
1.9
Tr5 |
1.5 |
C2
1.1
He+ |
1.0 0.9 |
|
C1 |
R1
α = 0.01 |
0.5 0.9 |
1.5 |
|
0.1 |
1.9 |
|
|
|
10 4 |
105 T, K |
Fig. 9.17. Phase diagram of helium. Triple points (for single and double ionization) are Tp3 and Tp4. Critical points of plasma phase transitions (for single and double ionization) are C1, C2. Shaded region represents the states obtained in experiments with multiple shock compression (Ternovoi et al. 2002).
9.3.4Sulfur
Sulfur is another substance used to study the dielectric–metal transition. The possibility of the transition was shown by Le Neindre et al. (1976), by extrapolating the shift of the absorption band edge to the high pressure range. Later on, this was verified by direct measurements of the electrical resistivity of the samples under pressure. The investigations were carried out both under static (Vereschagin et al. 1974; Chhabildas and Ruo 1977; Dunn and Bundy 1977; Evdokimova and Kuzemskaya 1978) and shock wave compression (David and Hamann 1958; Berger et al. 1960; Berg 1964; Yakushev et al. 1974; Nabatov et al. 1979). Naba-
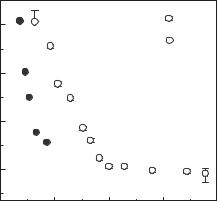
METALLIZATION OF DIELECTRICS |
359 |
r, mohm cm
109 |
–1 |
|
–2
107
105
103
0 |
50 |
100 |
150 P, GPa |
Fig. 9.18. Electrical resistivity of oxygen versus pressure. 1, multiple shock compres-
sion (Bastea et al. 2001); 2, single shock compression (Hamilton et al. 1988).
tov et al. (1979) used an experimental setup which provided the detection of the dielectric–metal transitions in the pressure range 100–200 GPa. The sulfur sample, together with the insulating teflon pads, was compressed between two copper screens. An impactor (accelerated by explosion) generated a shock with a rectangular profile in the screen. Since both sulfur and teflon are not as hard as copper, the maximum pressure corresponding to the pressure produced by the impactor on the screen was achieved by the shock reverberation between the screens. The resistivity of the sample was measured by employing the four–point circuit diagram. The shunt resistor was made of manganin foil and placed next to the sample, in order to diminish the parasitic inductance. In a plane parallel to the resistor, the manganin pressure sensor was installed, to be used for the shunt resistance measurements. Since no information about the sulfur equation of state in the high–pressure range was available, the resistivity ρ was calculated assuming that the sample was compressed to the maximum pressure by a single shock. The shock adiabat (Chhabildas and Ruo 1977) extrapolated up to 100 GPa was used for these estimates. The corresponding values of p and ρ are shown in Fig. 9.19 along with the data obtained by Berg (1964) at lower pressures with a single compression. One can see a fairly steep decrease of resistivity, followed by a transition to (approximately) constant value, ρ 10−2
The transition occurs at pressures about 19–20 GPa.
In order to analyze the qualitative influence of temperature on the resistivity, Postnov et al. (1986) decreased the temperature of the sample (with respect to the value corresponding to the shock adiabat) by employing multiple shock compression. For pressures below the transition value, ρ grows as the temperature decreases (by about three orders of magnitude), which is typical for semiconductors. Above the transition value, ρ weakly varies with temperature (decreases
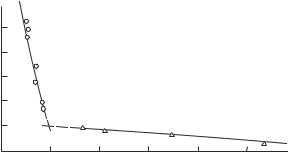
360 |
METALLIZATION OF NONIDEAL PLASMAS |
ρ, ohm cm.
p, GPa
Fig. 9.19. Resistivity of sulfur versus pressure: , multiple shock compression (Nabatov
et al. 1979), ◦, single shock compression (Berg 1964).
less than by a factor of two), which is typical for metals. This allows us to conclude that the dielectric–metal transition in sulfur occurs at p = 19–20 GPa, with electrons being the major charge carriers.
9.3.5Fullerene
In the crystalline state, fullerene is a semiconductor with an energy gap ∆E of about 2.1 eV and with rather narrow valence and conduction bands (about 0.5 eV each). The C60 molecules are mainly bound by the van der Waals forces, and the compressibility of C60 crystals is very high. One would expect that the valence and conduction band widths, which exponentially depend on the intermolecular distance, will rapidly increase with pressure. This process should be accompanied by a decrease in the energy gap, and, at some pressure, the crystal may transform to the metal state.
Analysis of the optical absorption spectra measured with C60 crystals at high pressure by Meletov et al. (1992) and Moshary et al. (1992) shows that the energy gap decreases rapidly with pressure. However, di erent estimates of the pressure corresponding to the transition to the metal state exhibit considerable scatter, from 20 to 70 GPa. Moreover, it is still unclear whether the transition of C60 crystals to the metal state is possible before the collapse of the molecules or their polymerization. The broad scatter of experimental data can be caused by several factors: First, high pressures may lead to the polymerization of C60 molecules. This is a rather slow process which can be present, to some extent, in all experiments and hence a ect the data for ∆E(p). Second, in most of the hydrostatic experiments a pressure-transmitting medium was used (such as liquid xenon, alcohol mixtures, etc.). Because of the large diameter of C60 molecules, the crystal structure is characterized by the presence of big intermolecular voids, which can be easily filled with the molecules of the pressure–transmitting medium. The latter process can strongly a ect the electron band structure of the samples.
METALLIZATION OF DIELECTRICS |
361 |
One can avoid the problems mentioned above by performing specially designed experiments with shock wave compression, which can provide a fairly smooth quasi–isentropic loading regime with minimal heating (Osip’yan et al. 2002). The idea of the method is as follows: The sample is placed between two plates made of glass or fused quartz. One plate is in contact with a metal screen. The dynamic loading occurs from the side of the screen, which is hit by a metal impactor accelerated by explosion products to a velocity of 2 km s−1. Because of the anomalous compressibility of glass and fused quartz below the elastic limit and by virtue of the thermodynamic laws governing the transition to the plastic state in these materials, no shock wave can exist at pressures below 12 GPa (Kanel’ and Molodets 1976). The shock wave is smeared our as it travels from the boundary between the quartz plate and the metal screen and transforms to a continuous wave of isentropic compression, which makes it possible to considerably reduce the irreversible shock heating of the sample. As a result, the sample is loaded smoothly – without a shock – under the conditions of the dynamic experiment. Earlier, this method had been successfully used by Fortov et al. (1999b, 2001) to study the “dielectrization” of lithium and sodium (see Section 9.3.7).
Using the dynamic compression method described above, the conductivity of the crystalline fullerene samples with density of 1.67 g cm−3 was studied under pressures of up to 20 GPa. It was found that, when the initial temperature was 293 K, the conductivity of the C60 samples reached values of σ 5 ohm−1cm−1, whereas for samples cooled to 77 K before the loading, the conductivity observed under a similar dynamic compression was almost two orders of magnitude smaller, σ 0.07 ohm−1cm−1. Note that in the first case, σ increases with pressure by seven to eight orders of magnitude with respect to the initial value. This kind of temperature dependence is typical for semiconductors and is similar to that observed in hydrogen, xenon, and oxygen. The results obtained by Osip’yan et al. (2002) suggest that the energy gap of C60 crystals decreases sharply with pressure. However, the gap does not decrease to zero, so that the samples compressed to a maximum pressure of 20 GPa remain semiconducting. Therefore, higher pressures are necessary in order to study the possibility of metallization in fullerene with the preserved molecular structure.
9.3.6Water
Water is one of the most abundant substances on the earth and in the universe. In particular, along with methane and ammonia it forms the main component of the inner shells of the giant planets in the solar system, Neptune and Uranus, occurring in the liquid phase at temperatures up to several thousand degrees and pressures up to hundreds of GPa. It is quite possible that the electrical conductivity of highly compressed and heated water is responsible for the magnetic field of these planets (Cavazzoni et al. 1999).
The physical properties of water under these extreme conditions have recently attracted detailed study. In particular, ab initio calculations of its phase diagram
362 |
METALLIZATION OF NONIDEAL PLASMAS |
using MD simulations by Cavazzoni et al. (1999) have shown that at pressures above 30 GPa and temperatures higher than 2000 K, water should be in a superionic state characterized by anomalously high proton mobility. The question of the predicted transition of water to the metallic state under the action of high pressures and temperatures (Cavazzoni et al. 1999) by analogy with molecular hydrogen is also fundamentally unresolved.
At present, the only source of experimental information on the properties of liquid water at high pressures and temperatures are experiments using powerful shock waves, which have already yielded unique data on its equation of state up to pressures of around 100 GPa by using explosives as the energy source (Bakanova et al. 1975), up to 230 GPa in experiments with light–gas guns (Mitchell and Nellis 1982), and at pressures of approximately 100 GPa (Volkov et al. 1980) and 1425 GPa (Podgurets et al. 1972) in experiments using underground nuclear explosions.
Results of the first measurements of the electrical conductivity of water beyond a shock wave front were obtained by David and Hamann (1959, 1960) who observed a sharp increase in conductivity as the dynamic pressure increased from 2 to 13 GPa and attributed this to an increase in the degree of dissociation of the water molecules to form ions. At about the same time Brish et al. (1960) made independent measurements of the conductivity at a dynamic pressure of 10 GPa and obtained a value of 0.2 ohm−1cm−1 in good agreement with the data of David and Hamann (1959, 1960). Somewhat later Hamann and Linton (1966) represented refined experimental values of the conductivity which were obtained at dynamic pressures up to approximately 22 GPa. Measurements of the electrical conductivity in aqueous solutions of KCl, KOH, and HCl in the range 7–13.3 GPa by Hamann and Linton (1969) allowed them to conclude that at pressures above 15–20 GPa the water beyond the shock wave front is almost completely dissociated to form ions and has an electrical conductivity of 10 ohm−1cm−1. The ionic nature of the electrical conductivity of dynamically compressed water is also supported by its optical transparency, observed by Zel’dovich et al. (1961) at pressures up to 30 GPa, and also by the good agreement between the dynamic and static (Holzapfel and Franck 1966) measurements of the electrical conductivity made at similar temperatures and pressures. Finally, using the two–stage light–gas gun, Mitchell and Nellis (1982) succeeded in making accurate measurements of the electrical conductivity of water at pressures of 25–59 GPa beyond the shock wave front.
Yakushev et al. (2000) substantially expanded the range of pressures to measure the electrical conductivity of water. This was accomplished by using a multistage dynamic loading regime in which the sample is exposed to the action of a series of successive shock waves circulating between two plane–parallel plates having a substantially higher dynamic rigidity than that of water. As a result, the water conductivity was measured up to pressures of 130 GPa and a density of 3.2 g cm−3. The ionic nature of the electrical conductivity of water under these experimental conditions was confirmed by recording the electrochemical