
- •Preface
- •Contents
- •1 Nonideal plasma. Basic concepts
- •1.1 Interparticle interactions. Criteria of nonideality
- •1.1.1 Interparticle interactions
- •1.1.2 Coulomb interaction. Nonideality parameter
- •1.1.4 Compound particles in plasma
- •1.2.2 Metal plasma
- •1.2.3 Plasma of hydrogen and inert gases
- •1.2.4 Plasma with multiply charged ions
- •1.2.5 Dusty plasmas
- •1.2.6 Nonneutral plasmas
- •References
- •2.1 Plasma heating in furnaces
- •2.1.1 Measurement of electrical conductivity and thermoelectromotive force
- •2.1.2 Optical absorption measurements.
- •2.1.3 Density measurements.
- •2.1.4 Sound velocity measurements
- •2.2 Isobaric Joule heating
- •2.2.1 Isobaric heating in a capillary
- •2.2.2 Exploding wire method
- •2.3 High–pressure electric discharges
- •References
- •3.1 The principles of dynamic generation and diagnostics of plasma
- •3.2 Dynamic compression of the cesium plasma
- •3.3 Compression of inert gases by powerful shock waves
- •3.4 Isentropic expansion of shock–compressed metals
- •3.5 Generation of superdense plasma in shock waves
- •References
- •4 Ionization equilibrium and thermodynamic properties of weakly ionized plasmas
- •4.1 Partly ionized plasma
- •4.2 Anomalous properties of a metal plasma
- •4.2.1 Physical properties of metal plasma
- •4.2.2 Lowering of the ionization potential
- •4.2.3 Charged clusters
- •4.2.4 Thermodynamics of multiparticle clusters
- •4.3 Lowering of ionization potential and cluster ions in weakly nonideal plasmas
- •4.3.1 Interaction between charged particles and neutrals
- •4.3.2 Molecular and cluster ions
- •4.3.3 Ionization equilibrium in alkali metal plasma
- •4.4 Droplet model of nonideal plasma of metal vapors. Anomalously high electrical conductivity
- •4.4.1 Droplet model of nonideal plasma
- •4.4.2 Ionization equilibrium
- •4.4.3 Calculation of the plasma composition
- •4.5 Metallization of plasma
- •4.5.3 Phase transition in metals
- •References
- •5.1.1 Monte Carlo method
- •5.1.2 Results of calculation
- •5.1.4 Wigner crystallization
- •5.1.5 Integral equations
- •5.1.6 Polarization of compensating background
- •5.1.7 Charge density waves
- •5.1.8 Sum rules
- •5.1.9 Asymptotic expressions
- •5.1.10 OCP ion mixture
- •5.2 Multicomponent plasma. Results of the perturbation theory
- •5.3 Pseudopotential models. Monte Carlo calculations
- •5.3.1 Choice of pseudopotential
- •5.5 Quasiclassical approximation
- •5.6 Density functional method
- •5.7 Quantum Monte Carlo method
- •5.8 Comparison with experiments
- •5.9 On phase transitions in nonideal plasmas
- •References
- •6.1 Electrical conductivity of ideal partially ionized plasma
- •6.1.1 Electrical conductivity of weakly ionized plasma
- •6.2 Electrical conductivity of weakly nonideal plasma
- •6.3 Electrical conductivity of nonideal weakly ionized plasma
- •6.3.1 The density of electron states
- •6.3.2 Electron mobility and electrical conductivity
- •References
- •7 Electrical conductivity of fully ionized plasma
- •7.1 Kinetic equations and the results of asymptotic theories
- •7.2 Electrical conductivity measurement results
- •References
- •8 The optical properties of dense plasma
- •8.1 Optical properties
- •8.2 Basic radiation processes in rarefied atomic plasma
- •8.5 The principle of spectroscopic stability
- •8.6 Continuous spectra of strongly nonideal plasma
- •References
- •9 Metallization of nonideal plasmas
- •9.1 Multiple shock wave compression of condensed dielectrics
- •9.1.1 Planar geometry
- •9.1.2 Cylindrical geometry
- •9.3 Metallization of dielectrics
- •9.3.1 Hydrogen
- •9.3.2 Inert gases
- •9.3.3 Oxygen
- •9.3.4 Sulfur
- •9.3.5 Fullerene
- •9.3.6 Water
- •9.3.7 Dielectrization of metals
- •9.4 Ionization by pressure
- •References
- •10 Nonneutral plasmas
- •10.1.1 Electrons on a surface of liquid He
- •10.1.2 Penning trap
- •10.1.3 Linear Paul trap
- •10.1.4 Storage ring
- •10.2 Strong coupling and Wigner crystallization
- •10.3 Melting of mesoscopic crystals
- •10.4 Coulomb clusters
- •References
- •11 Dusty plasmas
- •11.1 Introduction
- •11.2 Elementary processes in dusty plasmas
- •11.2.1 Charging of dust particles in plasmas (theory)
- •11.2.2 Electrostatic potential around a dust particle
- •11.2.3 Main forces acting on dust particles in plasmas
- •11.2.4 Interaction between dust particles in plasmas
- •11.2.5 Experimental determination of the interaction potential
- •11.2.6 Formation and growth of dust particles
- •11.3 Strongly coupled dusty plasmas and phase transitions
- •11.3.1 Theoretical approaches
- •11.3.2 Experimental investigation of phase transitions in dusty plasmas
- •11.3.3 Dust clusters in plasmas
- •11.4 Oscillations, waves, and instabilities in dusty plasmas
- •11.4.1 Oscillations of individual particles in a sheath region of gas discharges
- •11.4.2 Linear waves and instabilities in weakly coupled dusty plasmas
- •11.4.3 Waves in strongly coupled dusty plasmas
- •11.4.4 Experimental investigation of wave phenomena in dusty plasmas
- •11.5 New directions in experimental research
- •11.5.1 Investigations of dusty plasmas under microgravity conditions
- •11.5.2 External perturbations
- •11.5.3 Dusty plasma of strongly asymmetric particles
- •11.5.4 Dusty plasma at cryogenic temperatures
- •11.5.5 Possible applications of dusty plasmas
- •11.6 Conclusions
- •References
- •Index
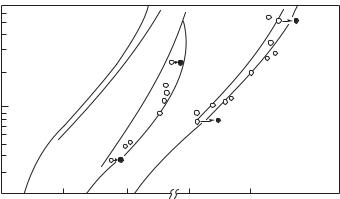
ON PHASE TRANSITIONS IN NONIDEAL PLASMAS |
229 |
. TPa
Fig. 5.30. Shock adiabats for aluminum and lead at ultrahigh pressures (Avrorin et al.
1987). Arrows indicate the shift of experimental data at the transition of the iron standard adiabat from curve 1 to curve 2.
Figure 5.31 illustrates the results of experiments by Trunin et al. (1969) with powerful shock–wave compression of porous copper enabling one to attain extremely high concentrations of thermal energy. At the maximum temperature of 2.5 · 105 K a superdense, quintuply ionized weakly degenerate (neλ3 0.7) plasma is obtained with extremely high parameters of p 2.09 TPa, ne 2 · 1023 cm−3, and Γ 2. Figure 5.31 demonstrates a reasonable description of the properties of such a medium with the “bound atom” model (5.77)–(5.83). We see that, as the Coulomb interaction decreases with the rise in temperature and pressure, the transition to a quasi–ideal multiply ionized plasma is realized. Yet the di erence between the experimental results (as well as Saha’s model (5.77)– (5.83)) and the Thomas–Fermi approximation increases gradually to reach, under extreme conditions, almost an order of magnitude in pressure, which supports the conclusion made by Iosilevskii and Gryaznov (1981) about the absence of the ideal–plasma asymptotes.
5.9On phase transitions in nonideal plasmas
Because of serious di culties in providing a consistent theoretical description of plasmas with strong interparticle interaction in the entire range of parameters, a number of researchers (see Wigner 1934; Norman and Starostin 1970; Ebeling et al. 1976; Zelener et al. 1981; Bushman and Fortov 1983; Redmer 1997; Likal’ter 2000; Iosilevskii and Starostin 2000, and references therein) make use of model consideration based on physical simplifications and extrapolations of views (developed for weakly nonideal plasmas) on the quantum and collective e ects under conditions of Coulomb interaction (Dharma-wardana and Perrot 1982). While being purely approximate, such a “heuristic” approach is conve-
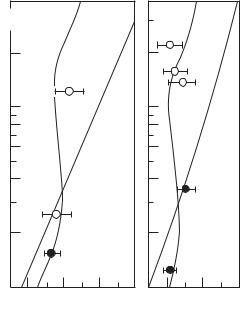
230 THERMODYNAMICS OF PLASMAS WITH DEVELOPED IONIZATION
. TPa
a |
b |
kg m
Fig. 5.31. Thermodynamics of nonideal copper plasma at extremely high pressures (Gryaznov et al. 1982). Initial porosity is m = ρ0/ρ00 = 3 (a) and m = 4 (b). Solid lines are shock adiabats calculated with the “bound” atom model (5.77)–(5.83); dashed lines are the Thomas–Fermi theory with corrections by Kalitkin and Kuzmina (1975, 1976);
◦ and • are experiments by Trunin et al. (1969) and Kormer et al. (1962), respectively.
nient and was widely used for qualitative analysis of the possible behavior of a plasma in situations characterized by the absence of measurements of its physical properties. Characteristically, a number of the proposed models in the region of increased nonideality lose thermodynamic stability, which is attributed to the possibility of a phase transition and the separation of the system into phases of di erent densities.
A model analysis (see Sections 5.1.4 and 10.2) of strongly compressed degenerate systems of electrons leads to the possibility of a phase transition with the formation of an ordered electronic structure (Wigner 1934) – a “Wigner crystal” (see Fig. 5.32). The properties of this crystal and its phase diagram have been studied in detail by numerous researchers (see, Carmi 1968; Norman and Starostin 1970; Norman 1971; Ebeling et al. 1976; Ceperley 1978; Maksimov and Dolgov 1982; Ichimaru 1992; Dubin and O’Neil 1999, and references therein) who considered the cases with zero and finite temperatures. In the latter case, the separation curve of an electron liquid and a Wigner crystal has a critical point while the stability limit of such a crystal at T = 0 is quite indeterminate
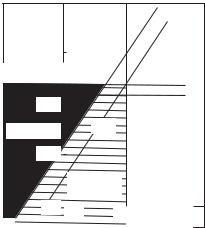
ON PHASE TRANSITIONS IN NONIDEAL PLASMAS |
231 |
cm |
|
Crystal |
Gas |
|
Classical |
|
region |
Fig. 5.32. Region of existence of the electron Wigner crystal (Wigner 1934; Ebeling et al.
1976): l = e2/kT
(see Section 5.1.4) (Carmi 1968; Norman 1971; Maksimov and Dolgov 1982). Ceperley 1978 related the phase transitions in OCP to the melting of metals. The dielectric permeability of strongly compressed Coulomb systems and the stability of a nonideal plasma were analyzed in reviews by Kirzhnits (1976) and Maksimov and Dolgov (1982).
More complex and diversified is the case of real multicomponent plasmas. Assuming that polarization is the principal e ect in a dense plasma, Mott (1967) and Ebeling et al. (1988) considered a model according to which the interatomic electrons are in the screened potential V (r) = −(e2/r) exp(−r/r0). The numerical solution of Schr¨odinger’s equation for this potential helps to find the critical value (Mott 1967) rc 0.84a0 = 0.84 2/me2, at which the ground state disappears and which corresponds to the so–called “Mott” metal–dielectric transition.
The possibility of anomalies in thermodynamic functions upon metallization of dense vapors was discussed by Zel’dovich and Landau 1944, who had assumed that metallic systems might have two interfaces, one for the liquid–gas transition and the other one for the metal–dielectric transition (see Fig. 5.33). The relation between these transitions and plasma anomalies was analyzed by Norman (1971).
There exists a substantial number of publications where the phase transformations in a nondegenerate, strongly nonideal plasma are investigated and numerous possibilities emerging in such plasmas are analyzed on the basis of simple models (Norman and Starostin 1970; Khrapak and Yakubov 1970; Ross and Greenwood 1971; Ebeling et al. 1976; Iosilevskii and Starostin 2000; Norman 2001). The principal e ect responsible for the condensation of a multicomponent plasma is the polarization attraction of opposite charges, whereas the stabilization of the emerging phase is due to the quantum e ects – interference and, at high densities, degeneracy and overlap of electronic shells of ions.
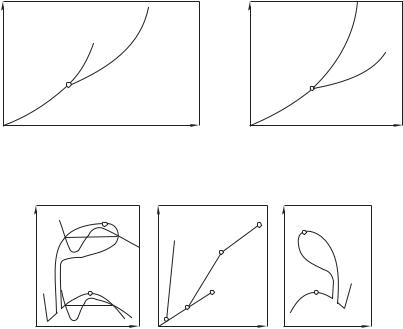
232 THERMODYNAMICS OF PLASMAS WITH DEVELOPED IONIZATION
MD
LG MD
LG
Fig. 5.33. Possible phase diagrams for metals (Zel’dovich and Landau 1944): LG – liq- uid–gas phase transition; MD – metal–dielectric transition
S |
DP |
|
|
M |
D |
|
|
L |
P |
G |
Sketches illustrating the possible behavior of phase equilibria (Norman 1971): a, b, c, c , d – critical and triple points; S – solid; L – liquid; G – gas; M – metal; D – dielectric; P – plasma; DP – dense plasma; 1,2 – isotherms.
Two phase transitions are predicted by the heuristic model of plasma metallization (Hansen et al. 1977). The determining factor at low densities and temperatures is the interatomic interaction leading to the gas–liquid transition (curve 1 in Fig. 5.34). With a density increase, the “ionization by pressure” occurs, along with a secondary violation of thermodynamic stability due to the Coulomb interaction. Therefore, the metal–dielectric transition in this model turns out to be the first–order phase transition which, as the temperature rises, changes over to a plasma phase transition (curve 2 in Fig. 5.34)
Among numerous calculations of the plasma phase boundaries, one should distinguish work by Ebeling et al. (1988) where the phase boundaries of a dense xenon plasma were derived. In these calculations, the Coulomb interaction was described by a broad–range Pad´e approximation (reproducing the asymptotes of Debye–H¨uckel and Hell–Mann–Brueckner), and the contribution of atoms and ions was described by the hard–sphere and Van der Waals models. It is demonstrated that this approach yields a first–order phase transition between weakly and strongly ionized states with the phase boundary of negative slope and the critical point at Tc 12 600 K, pc 9.9 GPa, and ρc 3.7 g cm−3. So far, experiments with xenon (see Section 5.8 and 9.3.2) in the vicinity of these boundaries

ON PHASE TRANSITIONS IN NONIDEAL PLASMAS |
233 |
gave no evidence of the phase transition. Possibly, additional measurements are required here.
Apart from the phase anomalies caused by the strong Coulomb interaction, possibilities are discussed of a phase transition in a weakly ionized plasma due to strong charge–neutral interaction (Khrapak and Yakubov 1970). One of the methods to estimate conditions for the dielectric–metal transition is based on a very old idea by Herzfeld 1927 of the so–called “dielectric catastrophe” (Maksimov and Shilov 1999). By employing the Clausius–Mossotti formula for the dielectric permittivity of a dielectric,
ε = 1 + |
4πnα |
, |
(5.98) |
1 − 4πnα/3 |
where n is the atom or molecule number density and α is the polarizability, one can expect the transition when ε diverges. This occurs at 4πnα/3 = 1. According to the simplest estimates by Edwards and Ashcroft (1997) based on the magnitude of α for a free hydrogen molecule, this condition is satisfied for p 150 GPa, which is in good agreement with other estimates performed with modern methods (Maksimov and Shilov 1999).
Being obviously approximate, the heuristic theories su er from a great uncertainty of predictions and often lead to qualitatively di erent conclusions. On the other hand, they serve as a permanent stimulus for the experimental search of these exotic e ects.
The hydrodynamic e ects accompanying dynamic action on a plasma in the presence of phase transitions can be detected upon shock or isentropic variation of pressure (Fortov 1972). In the latter case, the phase transition leads to an abrupt change of isentropic compressibility depending on the properties of competing phases. If this change is su ciently large, the rarefaction shocks are formed analogous to those recorded in iron and in ionic crystals (Altshuler 1965). In the general case, phase anomalies upon compression or expansion of metals would occur at the kinks of experimental and other thermodynamic curves discussed in Section 5.8. Especially characteristic in this respect are the experiments with the adiabatic expansion of shock–compressed metals (see Section 3.4), where in a single series of experiments one can follow the behavior of material over an extremely wide range of parameters and where one can expect to come across most of the phase anomalies predicted by theory (Figs. 5.35). The experimental data from isentropic compression and expansion of metals (see Figs. 3.17 and 3.18) as well as the data from rapid (Martynyuk 1977) and slow (Shaner and Gathers 1979) explosion of metals using an electric current have no clear hints of any anomalies which could be interpreted as phase transitions in nonideal plasma. Note that in the dynamic experiments one can detect both evaporation (Fig. 3.17 and 3.18) and melting (the latter produces little influence on the hydrodynamics of flow). In view of this, we would like to highlight studies by Ageev et al. (1988), where the optical measurements of the plasma temperature in an unloading wave also failed to reveal plasma anomalies.
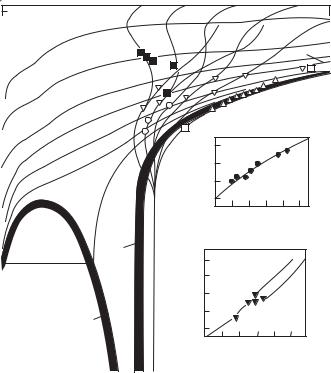
234 THERMODYNAMICS OF PLASMAS WITH DEVELOPED IONIZATION
Pa
. . . . .
.
km s
a
km s
b
Pa
g cm
Fig. 5.35. Phase diagram of copper (semiempirical equation of state from Bushman and Fortov (1988): m – di erent values of porosity at the shock adiabats; T – isotherms (in 103 K); M and R – phase boundaries of melting and boiling, respectively. The two–phase regions are shaded, dashed lines indicate the adiabats from Kormer et al. (1962). Insets show velocity of sound on the shock adiabats (a) and temperature in the unloading wave
(b).
Rusakov (1975) and Rusakov et al. (1977) presented the results of studies on the dynamics of expansion of shock–compressed materials in long (60–200 mm) cylindrical and cone–shaped channels. By measuring the velocities of luminescent plasma clusters and calculating the deviations from the results of self–similar solutions (obtained for an ideal gas with cp/cv = 1.4 on the assumption of stationarity of the incident wave), the authors draw the conclusion that phase anomalies are present in expanding plasma. Unfortunately, the plasma parameters in complex and obviously nonstationary flow were not measured directly, which forced the authors to make qualitative estimates. For instance, the density estimates were made assuming the plasma to be a noncompressible liquid, and the temperature estimates were based on the assumption of an ideal
ON PHASE TRANSITIONS IN NONIDEAL PLASMAS |
235 |
gas. According to Rusakov et al. (1977), the plasma temperature increases upon adiabatic expansion of the plasma.
Under the shock compression of a nonideal plasma, phase transitions would lead to a variation of shock compressibility whose increase, given some additional conditions (Fortov 1972), may cause the formation of continuous compression waves and multiwave structures, while a sharp decrease of compressibility may lead to the loss of shock wave stability. No such anomalies were revealed in experiments with the shock compression of cesium and heavy inert gases, and the recorded Hugoniot and Poisson adiabats (see Section 5.8) are smooth.
In addition, the experimentally found (see Sections 5.8 and 9.3) behavior of the variation of the thermodynamic functions of nonideal plasma corresponds to a one–phase case. Another indication of the absence of noticeable phase transformations in the range of parameters under investigation are the results of electrophysical (Chapter 7) and optical (Chapter 8) measurements, which can be well interpreted in the framework of one–phase plasma models.
Note that, based on existing knowledge (Fortov 1982; Bushman and Fortov 1983), phase transitions in dynamic experiments can occur over very short times (of the order of 10−10–10−6 s) which are considerably less than in the static case. This makes unlikely (though does not rule it out completely) the phase “quenching” under nonstationary conditions.
Numerous studies are devoted to the search for the electronic Wigner crystal in a solid–state plasma, with a thorough review of these studies being given by Tsidil’kovskii (1987). In common metals, the high electron number density (2 rs/a0 7) inhibits the formation of the Wigner crystal, so that the search was concentrated on highly compensated semiconductors and magnetic dielectrics.
By alloying n-type semiconductors with acceptors, one can substantially reduce the number density of free electrons and make the ion background close to uniform. In semiconductors of the type of ZnSb and Hg0.8Cd0.2Te, at n = nd − na 1014 cm−3 and na/nd 0.99, approximately 300 impurity ions are found inside the electron orbit, which makes the OCP model applicable. However, no clear proof of the Wigner condensation is obtained in this case, apparently because of the earlier Mott localization of electrons on donors, or because of the destructive e ect of fluctuating microfields.
In the case of magnetic isolators, the Wigner transition is apparently suppressed by the splitting of energy bands according to Hubbard’s model.
The presence of a threshold magnetic field in ZnSb – below which no activation energy was revealed in measurements of the Hall e ect and electrical conductivity – was treated as proof of the electron condensation. The results of these measurements are analyzed in detail by Tsidil’kovskii (1987), who produces arguments against the Wigner condensation, based on the fact that one deals with impurity electrons that are highly susceptible to the e ect of a random potential. Also, the speculations of Ceperley (1978) and about the electron condensation in crystals of n-Hg0.8Cd0.2Te are poorly grounded (Ceperley 1978; Ikezi et al. 1978), because apparently the electrons are localized on impurities
236 THERMODYNAMICS OF PLASMAS WITH DEVELOPED IONIZATION
due to the magnetic field.
Speaking about the plasma condensation, Norman and Starostin (1970), and Norman (1971) refer to a series of studies (Coock et al. 1955a, 1955b, 1981; Coock 1958; and Coock and McEwan 1958) which revealed “anomalous” e ects upon the arrival of a detonation wave from condensed explosive to the free surface of an explosive charge. Coock et al. (1955a,b, 1981); Coock (1958); and Coock and McEwan (1958) give an interpretation of the observed e ects, according to which a “Wigner crystal” is formed as a result of “chemical ionization” at the front of the detonation wave and, after this wave leaves the explosive charge, the crystal is in the metastable state. These plasma concepts formed the basis of the model of detonation of condensed explosives (Coock 1958), according to which the detonation-guiding process is the electron thermal conductivity rather than the shock wave.
A thorough analysis of the results of Coock et al. has shown (Davis and Campbell 1960; Fortov et al. 1974) that the proposed interpretation is not unambiguous and gives rise to objections, while the conclusions about the plasma phase transition lack substantiation, all the more that no direct measurements of density – which is the most a ected by such a transition – were performed by Coock et al. (as well as by Rusakov 1975 and Rusakov et al. 1977).
The exposure of plasma to pulse a X–ray radiation with a wavelength of 0.1 nm and 9 nm revealed (Fortov et al. 1974) no anomalously high plasma density, while the measured values proved to be eight to ten times higher than the free air density, this being in good agreement with the calculation of states behind the air shock wave front performed by Kuznetsov (1945) for a quasi–ideal gas approximation. Detection of the optical radiation emerging from a plasma has shown (Alekseev and Arkhipov 1962; Arkhipov 1965; Fortov et al. 1974) that, in contrast to the conclusions drawn by Coock et al., this radiation is not anomalous and corresponds to a brightness temperature of (8–10)·103 K, which is close to conventional calculations (Kuznetsov 1945). The optical absorption coe cient of the plasma also does not contain any qualitative anomalies and is described by the rarefied plasma model (see Chapter 7). Experiments involving detonation wave arrival into vacuum (Fortov et al. 1974) and helium (Davis and Campbell 1960) revealed no plasma glow, whereas, according to the concept of Coock et al., this glow must have intensified. In order to explain the observed velocities of the plasma clump, artificial reasoning about “reactive thrust during recombination” was used by Coock et al., while these velocities are easily calculated on the basis of the “discontinuity breakup” theory at the explosive–air interface.
Therefore, the performed experiments and analysis of available data demonstrate that the thermodynamic, optical, electrophysical, hydrodynamic and mechanical properties of the plasma clump are fully described by the ideal plasma model in the assumption that this clump is formed by compression and irreversible heating of air in the front of the wave emerging upon expansion of the detonation products. The wrong conclusion of Coock et al. about plasma condensation appears to be mainly due to the small velocity of the side expansion of the