
Учебники / Gene Therapy of Cochlear Deafness - Present Concepts and Future Aspects Ryan 2009
.pdf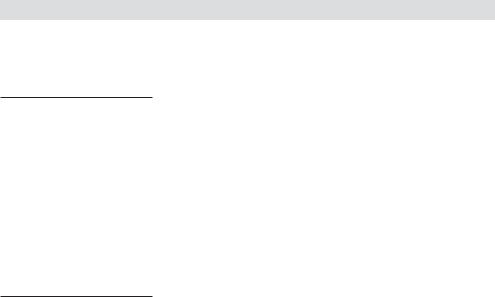
Ryan AF (ed): Gene Therapy of Cochlear Deafness.
Adv Otorhinolaryngol. Basel, Karger, 2009, vol 66, pp 64–86
Gene Therapy and Stem Cell Transplantation: Strategies for Hearing Restoration
Bradley W. Kessera Anil K. Lalwanib
aDepartment of Otolaryngology-Head and Surgery, University of Virginia School of Medicine, Charlottesville, Va., and bDepartment of Otolaryngology, New York University School of Medicine, New York, N.Y., USA
Abstract
Strategies to restore sensorineural hearing loss focus on the replacement of lost hair cells, the specialized mechanoreceptors in the organ of Corti that convert the mechanical energy of sound into electrical energy. Hair cells in mammalian systems do not have the capacity to regenerate, but two exciting lines of research hold promise in restoring inner ear function. Here we review basic principles of gene therapy and discuss its application in the inner ear. We survey the various viral vectors and routes of delivery into the inner ear. Applications of gene therapy in the inner include hair cell protection in the face of chemical or noise-induced ototoxicity, spiral ganglion cell survival following hair cell death or injury, and hair cell regeneration. More recently, the viability of gene therapy in human inner ear tissue has been reported. Transplantation of progenitor cells that can differentiate into functioning hair cells with the appropriate connections to their corresponding spiral ganglion cells is yet another strategy to restore sensorineural hearing loss. Neonatal or embryonic stem cells, adult mouse inner ear stem cells, and stem cells from the central nervous system have been shown to differentiate into cells containing hair cell markers and proteins. Prospects for stem cell therapy in the inner ear, and its limitations, will also be examined.
Introduction
With over 275 million people in the world affected, hearing loss is the most common sensory impairment in humans [http://www.who.int/pbd/deafness/en/]. Patients with sensorineural hearing loss, representing dysfunction in the cochlea or auditory nerve, far outnumber those with conductive type hearing losses. The most common site of lesion is the hair cell, a specialized mechanoreceptor located in the hearing organ (organ of Corti in mammals) and vestibular system of all vertebrates. For patients with sensorineural hearing loss, the causes are dysfunction, injury, or death of the hair cell. Through the displacement of stereocilia projecting from the apical surface of the cell, this highly specialized cell converts the mechanical energy of sound into
an electrical signal that is propagated along the auditory nerve to the brain, where the electrical energy is processed as sound perception.
In contrast to birds and lower vertebrates, hair cell loss is permanent in mammals. Hair cells do not regenerate, so that hearing loss from hair cell injury or loss is also permanent. Rehabilitation of sensorineural hearing loss currently consists of amplification devices (hearing aids) and devices that stimulate auditory neurons electrically (cochlear implants). Research into strategies to restore hearing following hair cell death has exploded in the last decade, and these approaches have generally taken one of three paths: (1) gene therapy designed to insert genes encoding functional proteins that can preserve, protect, or regenerate hair cells in the inner ear; (2) introduction of stem cells into the damaged cochlea, and (3) manipulation of genes controlling the cell cycle or regulating cell proliferation. This review focuses on the current state of gene transfer into the inner ear and on stem cell applications for hair cell recovery or renewal.
Basic Principles of Gene Transfer in the Inner Ear
Gene transfer (‘gene therapy’) refers to the infection (transfection) of cells in the inner ear with an engineered virus designed to drive expression of a functional protein. The expressed protein changes the function or behavior (phenotype) of the cell. The newly translated protein could be a transcription factor that induces new hair cell formation [1, 2], a missing protein in the complex hair cell apparatus (e.g., KCNQ4, a potassium protein whose genetic mutation has been linked to the autosomal dominant DFNA2) [3], a missing protein in the organ of Corti (e.g., connexin 26) [4], or a cytokine designed to protect the hair cell from acoustic or chemical injury (e.g., transforming growth factor-β1, TGF-β1, or glial cell line-derived neurotrophic factor, GDNF) [5, 6].
The potential advantages of gene transfer are the stable, long-term expression of the transgenic protein with the resultant long-term phenotypic change in the cell. Expression of the protein can be temporary or permanent; stable, long-term protein expression is paramount in cases of gene rescue or dominant negative suppression for genetic hearing loss. Cytokines designed to protect hair cells from injury can be infused directly into the cochlea, but their effects are transient, limited by the breakdown of the protein [7–11]. With the use of promoter sequences, gene transfer allows the cell to express the protective protein indefinitely or possibly to regulate protein expression. A second, short-term advantage of gene transfer is the use of viral vectors for experimental manipulations of endogenous proteins for the study of both normal physiology and pathophysiology of inner ear cells.
As the mechanisms underlying cochlear physiology and the genetic basis of hearing loss are discovered, a greater percentage of hearing loss – both age-related and congenital – will be attributed to genetic causes. Approximately 45 different ‘deafness
Inner Ear Gene Therapy and Stem Cell Transplantation |
65 |
genes’ have been identified for nonsyndromic hereditary hearing loss, and another 30 genes associated with syndromic hearing loss have been identified [for details, see the Hereditary Hearing Loss Homepage, http://webhost.ua.ac.be/hhh/], each of which is a potential target for gene transfer. Applications of gene transfer in genetic hearing loss include expression of exogenous proteins, gene rescue, or targeted gene replacement or gene silencing in cases of mutant phenotypes (e.g., connexin 26) and dominant negative or antisense suppression of endogenous protein function [12].
By contrast, in cases of acquired hearing loss, gene transfer holds promise for the protection of hair cells in the face of ototoxic insult, including chemical and noiseinduced trauma [5, 6, 13], rescue of hair cells after ototoxic injury [14], preservation and protection of spiral ganglion neurons after hair cell loss [15–18], and hair cell regeneration [1, 2].
The inner ear has proven to be an ideal system for studies in viral-mediated gene transfer for several reasons. The bony housing of the sensory epithelium prevents, or at least limits, unwanted systemic viral diffusion. While egress from the guinea pig cochlea via a presumed patent cochlear aqueduct is hypothesized to cause transduction of the contralateral cochlea and CSF, the heart, lung, liver, spleen, or kidney were not transfected as these vectors do not gain access to the systemic circulation nor do they transfect solid organ systems presumably due to the integrity of the blood-brain and blood-labyrinthine barriers [19–21]. The human cochlear aqueduct is closed, so engineered viruses and their genetic payloads cannot diffuse into surrounding tissue and cause unintended injury.
The low volume, fluid-filled chambers of the inner ear are in continuity, allowing the spread of virus throughout all regions of the inner ear. Nevertheless, numerous studies show a gradient of transfection when virus is inoculated into the scala tympani via the round window: greatest at the inoculation site (basal end of cochlea) and least at the apex for all vectors studied, including adenovirus [22], cationic liposome [22, 23], HSV-1, and vaccinia virus vectors [24]. An important consideration for future studies will be the uniform distribution of virus throughout the entire cochlea (or vestibular labyrinth).
Methods of Gene Delivery
An overarching theme in studies examining viral delivery methods has been the suboptimal expression of the viral transgene with techniques that better preserve hearing. Protocols that violate the otic capsule of the cochlea result in greater threshold shifts on auditory brainstem responses (ABRs). For hearing preservation, drug delivery techniques to the human inner ear are limited to intratympanic injections; inoculation of a viral vector through the tympanic membrane into the middle ear of the neonatal rat preserved auditory and vestibular function but did not result in reporter gene expression in the cochlea or vestibular system [25]. A gelatin sponge soaked
66 |
Kesser · Lalwani |
with adenovirus and liposome, but not adeno-associated virus (AAV), was successful in transducing cochlear tissues through an intact round window membrane in the mouse with preservation of hearing as measured by ABR testing [22]. Low transfection rates of supporting cells and hair cells in the organ of Corti have been the criticism of these ‘cochlea-sparing’ techniques.
Comparison of vector delivery methods using an advanced generation adenoviral vector showed expression of the GFP reporter gene in the cochlea with both round window membrane inoculation and basal turn cochleostomy inoculation, with preservation of hearing in the round window group and high frequency hearing loss in the cochleostomy group [26, 27]. Canalostomy in the posterior semicircular canal of the mouse preserved hearing, but reporter gene expression was limited to the cells lining the perilymphatic space. Cochleostomy inoculation in the same study showed expression of the reporter gene in the sensory cells of the saccule and organ of Corti, but these animals showed significant threshold shifts on ABR testing [28].
Viral inoculation in the guinea pig endolymphatic sac resulted in reporter gene expression in the sac, the endolymphatic duct, the vestibular end organs (no hair cells infected), and in the cochlea, specifically, cells lining the fluid spaces, marginal cells of the stria vascularis, endothelial cells in Reissner’s membrane, and Hensen’s cells in the organ of Corti [29]. Inoculation of an HSV-1 vector through a small opening in the utricle also preserved hearing while achieving efficient reporter gene expression [30].
Efficient delivery of viral (or non-viral) vectors to the inner ear requires a technique that delivers an appropriate volume of vector with equal distribution throughout the cochlea or vestibular labyrinth while preserving hearing function, cochlear architecture, and the carefully balanced ionic gradients in the inner ear. For human application, the technique must be safe with minimal risk to the patient.
Viral Vectors
Engineered replication-deficient viruses including lentivirus [31], adenovirus [32– 34], herpes simplex type 1 virus [24, 35], vaccinia virus [24], AAV [19], and a novel bovine adeno-associated viral vector [36] have been shown to transfect varying cell types in the nonprimate mammalian inner ear. Each of these viral vectors has its own profile with regard to the specific inner ear tissues it selectively transfects, stability of transfection, toxicity, genetic carrying capacity, ability to integrate into the host genome, and host inflammatory reaction (table 1).
Adenovirus
A linear 36-kbp dsDNA virus, adenovirus infects a wide variety of organ systems, tissue types, and cells in humans, a distinct advantage for manipulation in the laboratory. Adenovirus does not require cell division for transduction, another significant
Inner Ear Gene Therapy and Stem Cell Transplantation |
67 |
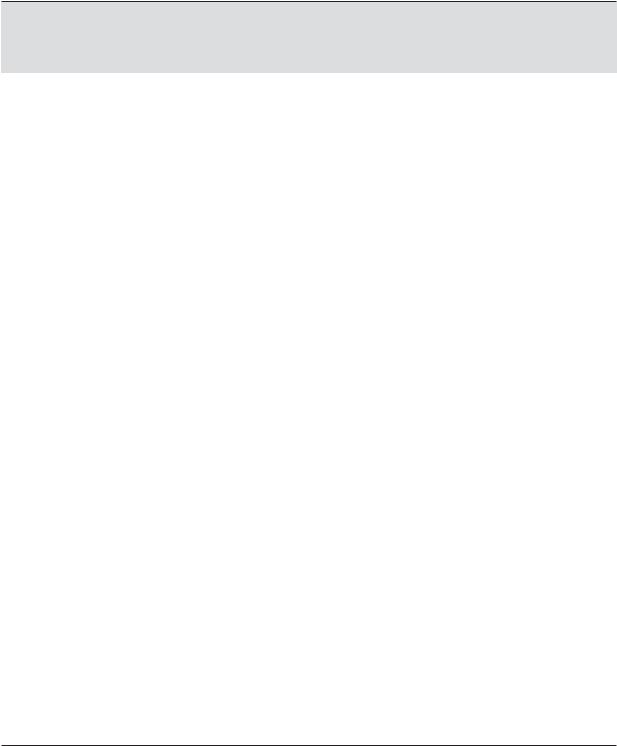
Table 1. Viral vectors: advantages, disadvantages, and cellular tropism
Viral vector |
Advantages |
Disadvantages |
Cellular tropism |
References |
|
|
|
(based on |
|
|
|
|
expression of |
|
|
|
|
reporter genes) |
|
|
|
|
|
|
Adenovirus |
Easy to produce |
Immunogenic |
Mesenchymal cells |
Raphael et al. [32], 1996 |
|
Large (7.5–10 kb) |
Transient expression |
lining perilymphatic |
Dazert et al. [33], 1997 |
|
insert |
|
spaces |
Holt et al. [34], 1999 |
|
Cell division not |
|
Stria vascularis |
Dazert et al. [25], 2001 |
|
required |
|
Fibrocytes of spiral |
|
|
|
|
ligament |
|
|
|
|
Supporting cells of OC |
|
|
|
|
Hair cells of OC and |
|
|
|
|
Vestib |
|
|
|
|
SGNs |
|
|
|
|
|
|
Adeno-associated |
No human disease |
Difficult to produce |
Fibrocytes of SL |
Lalwani et al. [19], |
virus |
Stable expression |
Small (4–5 kb) insert |
Rm |
1996 |
|
Cell division |
Variable transfection |
Supporting cells of OC |
Lalwani et al. [123], |
|
not required |
efficiency |
SGNs |
1997 |
|
|
|
Vestibular sensory hair |
Lalwani et al. [48], |
|
|
|
cells and supporting |
1998 |
|
|
|
cells |
Lalwani et al. [49], |
|
|
|
|
1998 |
|
|
|
|
|
Herpesvirus |
Large (10–100 kb) |
Human disease |
Mesenchymal cells |
Derby et al. [24], 1999 |
|
insert |
Cytopathic |
lining perilymphatic |
Geschwind et al. [35], |
|
Stable expression |
|
spaces |
1996 |
|
|
|
Fibrocytes of SL |
|
|
|
|
Rm |
|
|
|
|
Supporting cells of OC |
|
|
|
|
SGNs; VGNs |
|
|
|
|
|
|
Lentivirus |
Stable expression |
Insertional |
SGNs |
Han et al. [31], 1999 |
(retrovirus) |
|
mutagenesis |
Glia |
|
|
|
Low transfection |
|
|
|
|
efficiency |
|
|
|
|
Cell division required |
|
|
|
|
|
|
|
Liposome |
Easy to make |
Transient expression |
Fibrocytes of SL |
Wareing et al. [23], |
|
Unlimited insert size |
Low transfection |
OHCs and SCs of OC |
1999 |
|
Cell division not |
|
Rm |
|
|
required |
|
SGCs |
|
|
Nonpathogenic; |
|
|
|
|
nonimmunogenic |
|
|
|
|
|
|
|
|
OC = Organ of Corti; SGNs = spiral ganglion neurons; SL = spiral ligament; Rm = Reissner’s membrane; VGNs = Vestibular ganglion neurons; OHCs = outer hair cells; SCs = supporting cells. Adapted from Lalwani and Mhatre [53], Staecker et al. [124] and Holt [12].
68 |
Kesser · Lalwani |
advantage for transfecting the post-mitotic terminally differentiated cells of the mammalian inner ear. Intracellularly, adenovirus acts as an episome and does not integrate into the host genome, eliminating the risk of insertional mutagenesis at the expense of a more transient expression.
Exogenous genes can be inserted into the adenoviral genome using a strategy of homologous recombination in bacteria, and high titers of virus are easily obtained [37]. Adenovirus can carry a large genetic payload, with insertional genes as large as 10 kbp. With the cytomegalovirus promoter, generally high rates of transgene expression can be achieved. While early studies using E1–, E3–-deleted adenoviral vectors showed substantial dose-dependent and time-dependent hair cell toxicity [33, 34, 38], more recent studies using a modified multiply deleted (E1– , E3– , pol– pTP–) adenovirus [12] preserved cochlear function as measured by distortion-product otoacoustic emissions [39] and by hair cell transduction [12, 40] without compromising viral infectivity.
Given the numerous candidate viral vectors that have been studied, adenovirus is a particularly attractive vector for inner ear gene transfer [for review see, 41, 42] because it has been shown to efficiently transduce both neural and glial cells of the mouse central nervous system [43] and, in the inner ear, a broad spectrum of cell types including hair cells and supporting cells [28, 39, 44–46]. More recently, adenovirus has been shown to transfer exogenous genetic material into both hair cells and supporting cells of cultured human vestibular epithelia, furthering its attractiveness as a vehicle for gene transfer into the human inner ear [47] (fig. 1).
Adeno-Associated Virus
A 4.5-kbp single-stranded DNA parvovirus, AAV transduces preand post-mitotic cells and does not require actively dividing cells for transfection. It does require, however, adenovirus or herpes simplex virus (HSV) for autonomous replication. Unlike adenovirus, AAV is thought to incorporate into the host genome resulting in stable, potentially long-term, transgenerational expression. Such long-term expression could potentially substitute for a specific gene dysfunction within the cochlea, e.g., connexin 26 mutation. AAV is safe and not associated with human disease or infection. Because it is a relatively small virus, AAV carries a smaller genetic ‘payload’ and thus has limitations when considering transfection of larger genes.
AAV (serotype 2) drives expression of genes into many cell types of the inner ear, including spiral ganglion cells (SGCs), cells of the spiral limbus and spiral ligament [19, 48], sensory and supporting cells of the cristae ampullaris and maculae of mammalian vestibular organs [49], and hair cells and supporting cells of murine cochlea explants [50] (fig. 2). Stable expression for periods up to 24 weeks in the mammalian inner ear [48] and 6 months in adult rat striatal neurons has been observed [51]. Interestingly, AAV gene expression has been found to be serotypeand promoterdependent [38, 50] leading to the conclusion that cell-specific transgene expression can be established by using specific serotypes and promoters in AAV constructs [50].
Inner Ear Gene Therapy and Stem Cell Transplantation |
69 |
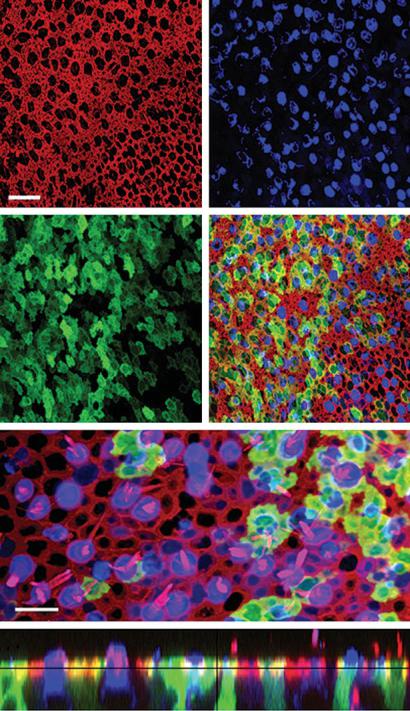
Fig. 1. Confocal images of a human utricle harvested from a patient undergoing labyrinthectomy for Ménière’s disease. The explant was exposed to 107 viral particles/ml of Ad2-GFP for 15 h and maintained in culture for 48 h. The explant was then fixed and stained with Alexa Fluor 546-conjugated phalloidin and an antibody to myosin 7a and an Alexa Fluor 633-conjugated secondary antibody. a An image of the apical surface of the epithelium shows the actin (red) pericuticular necklace that rings each hair cell and supporting cell. Scale bar = 20 μm and applies to a–d which show the same image plane. b Myosin 7a immunoreactivity (blue) localizes sensory hair cells. c Green fluorescence revealed that both hair cells and supporting cells were transfected by the vector and expressed GFP. d Merge of panels a–c. e Higher magnification overlay view from a different region of the same epithelium shown in panels (a–d). To generate the image a stack of 13 images focused at the hair bundle level was collapsed (red = actin) and overlaid atop a collapsed stack of 10 images focused at the cell body level from the same image field (blue = myosin 7a; green = GFP). Scale bar = 10 mm. f A stack of 19 images projected to reveal a crosssectional view of the sensory epithelium. Both type I (HC1) and type II hair cells (HC2) and supporting cells were GFP positive. Myosin 7a-positive hair cells are also evident. Same color code as for panels a–d. Reprinted from Kesser et al. [47].
a |
b |
c |
d |
e
f
HC1 |
HC2 |
HC2 |
SC |
HC2 |
SC |
HC1 SC |
HC2 |
70 |
Kesser · Lalwani |

Stria vascularis
|
Reissner’s |
Scala vestibuli |
membrane |
Scala media
Spiral limbus
Hair cells
Supporting cells
Spiral ligament
Auditory neurons
Adenovirus and AAV
Adenovirus alone
Scala Tympani
Fig. 2. Schematic representation of the cellular tropism of adenoviral vector and adeno-associated virus (AAV) for cells in the mammalian cochlea. Printed with permission from Anil K. Lalwani, MD.
Herpes Simplex Virus
A double-stranded 152-kbp DNA virus, HSV infects a broad range of cell types including epithelial cells, fibroblasts, and neurons. The most commonly used vector is derived from herpes simplex type 1 (HSV-1), and these HSV-1 vectors effectively deliver foreign genes to the post-mitotic cells of the mammalian nervous system. With its large genome, HSV-1 can be engineered to carry single or multiple, large genetic inserts. The vector can infect dividing or post-mitotic cells, and its ability to assume a latent state in neurons represents a significant advantage that could potentially mediate stable, long-term transgene expression. A latent stage HSV-1 virus in a host neuronal cell is resistant to host immune surveillance. The virus does not incorporate into the host genome and exists as an episome in the neuronal nucleus, eliminating the possibility of insertional mutagenesis.
Inner Ear Gene Therapy and Stem Cell Transplantation |
71 |
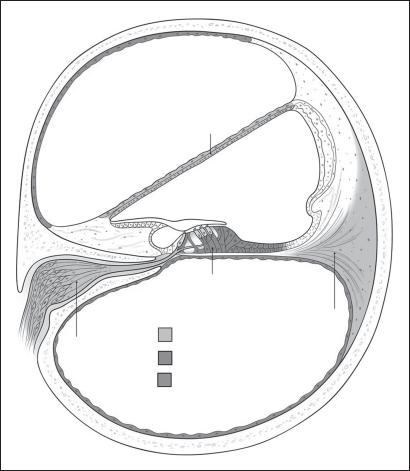
Scala vestibuli |
Reissner’s |
membrane |
Scala media
Auditory neurons*
*Lentivirus in vitro, not in vivo
Supporting cells
Spiral ligament
Herpes virus and Lentivirus
Herpes virus alone
Lentivirus alone
Scala Tympani
Fig. 3. Schematic representation of the cellular tropism of herpesvirus vectors, lentiviral vectors for cells in the mammalian cochlea. Printed with permission from Anil K. Lalwani, MD.
In the inner ear, HSV-1 vectors infect fibrocytes of the spiral ligament, mesenchymal and epithelial cells of Reissner’s membrane, Hensen’s cells and Deiter’s cells in the organ of Corti, and mesenchymal cells lining the scala vestibuli when inoculated via a micropipette inserted through the round window (fig. 3); hair cells were not transduced [24]. Lack of hair cell transfection by HSV-1 was confirmed by Staecker et al. [52] who observed expression of the marker protein β-galactosidase in auditory and vestibular neurons, in cells in the stria vascularis, and in the supporting cells of the organ of Corti. While very little cytotoxicity and T-cell immune response (limited to the site of inoculation) of an HSV-1 vector were noted in one study [52], a brisk lymphocytic infiltration of the scala tympani was observed in all turns of the cochlea in another study [24].
72 |
Kesser · Lalwani |
Given its tropism for neurons, HSV-1 may prove to be an effective vector for gene transfer of neurotrophic factors into the SGCs of the cochlea, and may prevent SGC degeneration and promote neuronal survival after acquired hair cell injury or loss [17, 24]. Limitations of HSV-1 include difficult production, low infection efficiency, and its potential immunogenicity/cytotoxicity [53].
Others
Vaccinia virus, the live virus used in the smallpox vaccine, has had limited use in studies of inner ear gene transfer, mostly because of the brisk inflammatory response observed in the basal two turns of the cochlea in animals injected with a vaccinia virus vector [24]. Retroviruses are single-stranded RNA viruses containing a reverse transcriptase that directs synthesis of a double-stranded DNA molecule that enters the cell nucleus for incorporation into the host genome. This integration of viral DNA into the host genome requires cell division, so that retroviral vectors can only integrate into the genome of dividing cells. While retroviral vectors have generated significant interest in their ability to target rapidly dividing neoplastic cells [for review see, 54–56], they are not considered efficient vectors for gene transfer into the postmitotic cells of the inner ear.
Lentivirus, derived from the human immunodeficiency virus (HIV) is a retroviral vector that can infect nondividing cells [57]. Lentiviral vectors infect a broad range of both dividing and nondividing cells, including the terminally differentiated neuron; lentivirus-mediated protein expression has been demonstrated in the rat brain without observable toxicity for as long as 6 months [58, 59]. The ability of lentiviral vectors to transduce post-mitotic cells and to integrate into the host genome makes them potentially excellent candidates for long-term, stable protein expression in the inner ear, a significant advantage in the treatment of genetic hearing loss. Although lentivirus transgene expression in vivo in the inner ear was low (1–3%) and restricted to cells along the periphery of the perilymphatic space and spiral ligament, in vitro lentivirus has been shown to transfect spiral ganglion neurons [31] (fig. 3). The limited expression seen with lentivirus in vivo reflects more its distribution not its true ability to infect the variety of cells within the cochlea. Lentivirus may be a suitable vector for the secretion of neurotrophins and other protective factors as gene products into the perilymph.
To avoid problems of immunogenicity, insertional mutagenesis, and viral vectorrelated disease or toxicity, vesicles composed of a bilayer of lipid molecules enclosing an aqueous volume have been complexed with DNA to produce cationic liposomes as drug or gene delivery vectors [60]. Liposomes bind to the plasma membrane where DNA material is internalized. The bound DNA does not replicate or integrate into the host genome. These liposomes have the advantage of an unlimited genetic carrying capacity and simplicity of production. Nevertheless, they have proven to be inefficient vectors to transfer exogenous DNA, with essentially no control over the target cell other than the site of vector delivery [52]. While cationic liposomes have been
Inner Ear Gene Therapy and Stem Cell Transplantation |
73 |