
Учебники / Gene Therapy of Cochlear Deafness - Present Concepts and Future Aspects Ryan 2009
.pdf
Table 2. In vivo gene transfer to the mammalian inner ear
Animal |
Delivery route |
Expression in |
Vector |
Dose |
Duration |
Others |
Reference |
|
|
cochlea |
|
|
|
|
|
|
|
|
|
|
|
|
|
Mouse |
Lateral |
Sensory cells in |
Ad.CMV-lacZ |
8 ×1011 pfu/ |
28 days |
|
Kawamoto |
|
canalostomy |
crista ampullaris |
(β-galactose |
ml, 0.5–1.0 |
|
|
et al. [58] |
|
|
|
gene), |
min |
|
|
|
|
|
|
serotype5 |
|
|
|
|
|
|
|
(E1–, E3–) |
|
|
|
|
|
Cochleostomy |
Inner hair cells, |
|
|
|
ABR |
|
|
|
Deiter’s cells, scala |
|
|
|
threshold |
|
|
|
tympani, Reissner’s |
|
|
|
increase 13 |
|
|
|
membrane |
|
|
|
days after |
|
|
|
|
|
|
|
injection |
|
|
|
|
|
|
|
|
|
Neonatal |
Cochleostomy |
Scala tympani, |
Ad.CMV-lacZ |
5×106 pfu/ |
7 days |
|
Ying et al. |
rat (P5) |
|
Reissner’s |
(E1–) |
ml, 2 min |
|
|
[105] |
|
|
membrane, organ |
|
|
|
|
|
|
|
of Corti (sporadic) |
|
|
|
|
|
|
|
|
|
|
|
|
|
Guinea pig |
Cochleostomy |
|
Ad.CMV-lacZ |
5×108 pfu/ |
8 days |
DPOAE lost |
Luebke et al. |
|
|
|
(E1–, E3–) |
cochlea, |
|
|
[71] |
|
|
|
|
infusion |
|
|
|
|
|
Inner and outer |
Ad.CMV-lacZ |
5×108 pfu/ |
|
DPOAE |
|
|
|
hair cells |
(E1–, E3–, pol–) |
cochlea, |
|
intact |
|
|
|
|
|
infusion |
|
|
|
|
|
|
|
|
|
|
|
Guinea pig |
RWM damaged |
Spiral limbus, spiral |
Ad.lacZ |
1×109 p/ml, |
3 days |
|
Suzuki et al. |
|
by local |
ligament, inner |
|
20 min |
|
|
[76] |
|
anesthetics |
and outer hair |
|
|
|
|
|
|
|
cells, supporting |
|
|
|
|
|
|
|
cells, spiral |
|
|
|
|
|
|
|
ganglion, scala |
|
|
|
|
|
|
|
tympani |
|
|
|
|
|
|
|
|
|
|
|
|
|
Guinea pig |
Endolymphatic |
Reissner’s |
Ad.CMV-lacZ, |
1×1010 p/ |
4 days |
|
Yamasoba |
|
sac |
membrane, |
serotype 5 |
ml,10–15 |
|
|
et al. [67] |
|
Widening of |
marginal cells of |
(E1–, E3–) |
min |
|
|
|
|
endolymphatic |
stria vascularis, |
|
|
|
|
|
|
duct |
spiral ligament, |
|
|
|
|
|
|
|
supporting cells |
|
|
|
|
|
|
|
(endolymphatic |
|
|
|
|
|
|
|
duct, transitional |
|
|
|
|
|
|
|
epithelium |
|
|
|
|
|
|
|
surrounding the |
|
|
|
|
|
|
|
sensory area of |
|
|
|
|
|
|
|
utricle and saccule) |
|
|
|
|
|
|
|
|
|
|
|
|
|
24 |
Maeda · Sheffield · Smith |
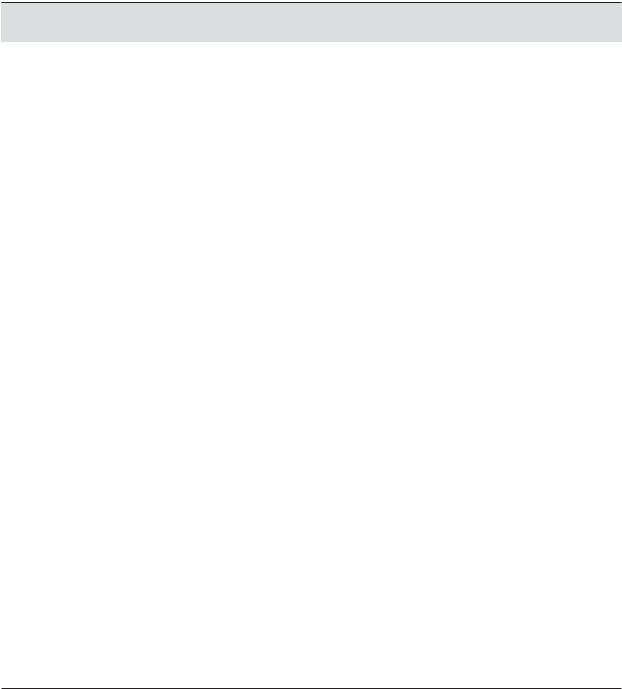
Table 2. Continued
Animal |
Delivery route |
Expression in |
Vector |
Dose |
Duration |
Others |
Reference |
|
|
cochlea |
|
|
|
|
|
|
|
|
|
|
|
|
|
Guinea pig |
Cochleostomy |
Inner hair cells, pillar Ad.CMV-lacZ, |
5×108 pfu/ |
8 days |
DPOAE lost |
Christoph |
|
|
|
cells |
serotype 5 |
cochlea, |
|
|
et al. [81] |
|
|
|
(E1–, E3–) |
infusion |
|
|
|
|
|
No expression |
AAV. CMV-lacZ, |
5×108 i.p./ |
8 days |
DPOAE intact |
|
|
|
|
promoter |
cochlea, |
|
|
|
|
|
|
|
infusion |
|
|
|
|
|
Nerve fibers |
NSE promoter |
|
|
|
|
|
|
Spiral limbus |
PDGF promoter |
|
|
|
|
|
|
Epithelium of blood EF-1α promoter |
|
|
|
|
|
|
|
vessels |
|
|
|
|
|
|
|
No expression |
β-actin |
|
|
|
|
|
|
|
promoter |
|
|
|
|
|
|
|
|
|
|
|
|
Guinea pig |
Rupture of |
Spiral ganglion, |
Ad.RSV-lacZ |
1×1011– |
56 days |
|
Li Duan et al. |
|
RWM |
Reissner’s |
(E1–, E3–) |
2×1011 |
|
|
[74] |
|
|
membrane, |
|
pfu/ml |
|
|
|
|
|
epithelial cells in |
|
|
|
|
|
|
|
basilar membrane |
|
|
|
|
|
|
|
Spiral ganglion, |
AAV.CMV-GFP |
1.5×108 |
28 days |
|
|
|
|
stria vascularis |
|
i.p./ml |
|
|
|
|
|
|
|
|
|
|
|
Guinea pig |
Cochleostomy |
Spiral limbus, spiral |
AAV.lacZ AAV. |
1×105 i.p./ |
14 days |
|
Arnold et al. |
|
|
ligament Reissner’s |
CMV-GFP |
cochlea, |
|
|
[80] |
|
|
membrane, organ |
|
infusion |
|
|
Lalwani et al. |
|
|
of Corti, spiral |
|
|
|
|
[60] |
|
|
neurons |
|
|
|
|
|
|
|
|
|
|
|
|
|
Guinea pig |
Cochleostomy |
Scala tympani, |
VSV |
1×107 p/ml, |
14 days |
|
Han et al. [64] |
|
|
scala vestibuli |
G-pseudotyped |
infusion |
|
|
|
|
|
|
|
|
|||
|
|
|
LV.CMV-GFP |
|
|
|
|
|
|
|
|
|
|
|
|
Guinea pig |
Rupture of |
Spiral ligament, |
HSV-1.lacZ |
6×109 pfu/ |
4 days |
Reduction |
Derby et al. |
|
RWM |
Reissner’s |
|
ml, 2 μl |
|
in gene |
[73] |
|
|
membrane |
|
|
|
expression |
|
|
|
Supporting cells |
|
|
|
after 6 days |
|
|
|
Scala vestibuli |
|
|
|
|
|
Spiral ligament, Reissner’s membrane, inner and outer hair cells, scala vestibuli, scala tympani
RNA Interference in the Inner Ear |
25 |

Table 2. Continued
Animal |
Delivery route |
Expression in |
Vector |
Dose |
Duration |
Others |
Reference |
|
|
cochlea |
|
|
|
|
|
|
|
|
|
|
|
|
|
Guinea pig |
Rupture of |
Spiral ligament, |
Liposome- |
0.2 μg/μl |
14 days |
|
Wareing et al. |
|
RWM |
spiral limbus, |
plasmid.CMV- |
10 μl |
|
|
[72] |
|
|
organ of Corti, |
βgal |
(injection) |
|
|
|
|
|
Reissner’s |
|
or infusion |
|
|
|
|
|
membrane, spiral |
|
|
|
|
|
|
|
neurons |
|
|
|
|
|
|
|
|
|
|
|
|
|
Mouse |
Intact RWM |
Spiral limbus, |
Liposome- |
0.2 μg/μl |
3 days |
|
Jero et al. |
|
|
Reissner’s |
plasmid.CMV- |
|
|
|
[65] |
|
|
membrane, |
hrGFP |
|
|
|
|
|
Cochleostomy |
spiral neurons |
Liposome- |
|
|
|
|
|
|
|
|
|
|
||
|
|
|
plasmid.CMV- |
|
|
|
|
|
|
|
hrGFP |
|
|
|
|
|
|
|
|
|
|
|
|
Mouse |
Intact RWM |
Spiral ligament, |
Liposome- |
0.5 μg /μl, |
3 days |
|
Jero et al. |
|
|
spiral limbus, organ |
plasmid.CMV- |
2μl |
|
|
[66] |
|
|
of Corti, Reissner’s |
eGFP |
|
|
|
|
|
|
membrane, spiral |
|
|
|
|
|
|
|
neurons |
|
|
|
|
|
|
|
Scala tympani, |
Ad.CMV-eGFP |
6×1010 pfu/ |
7 days |
|
|
|
|
Reissner’s |
|
ml, 2 μl |
|
|
|
|
|
membrane |
|
|
|
|
|
|
|
No expression |
AAV.CMV-GFP, |
2×1011 pfu/ |
7 days |
|
|
|
|
|
AAV.CMV-lacZ |
ml, 2 μl |
|
|
|
|
|
|
|
|
|
|
|
Mouse |
Intact RWM |
Spiral limbus, spiral |
Liposome- |
0.5 μg/μl, 5 |
3 days |
|
Maeda et al. |
|
|
ligament, outer |
plasmid.CMV- |
μl |
|
|
[2] |
|
|
hair cells, inner and |
eGFP |
|
|
|
|
|
|
outer pillar cells |
|
|
|
|
|
|
|
|
|
|
|
|
|
Mouse |
Rupture of |
Stria vascularis, |
Ad.CMV-GFP |
1×108 |
3 days |
|
Staecker et al. |
|
RWM |
spiral ligament, |
|
–1×1010 |
|
|
[70] |
|
|
outer hair cells, |
|
pfu/ml,1 μl |
|
|
|
|
|
Dieter’s cells |
|
|
|
|
|
|
|
Stria vascularis, |
HSV-1.CMV-lacZ |
2×107,1×108 |
|
|
|
|
|
supporting cells, |
|
pfu/ml, 1 μl |
|
|
|
|
|
spiral neurons |
|
|
|
|
|
|
|
Stria vascularis, |
Liposome- |
|
|
|
|
|
|
spiral neurons, |
plasmid.CMV- |
|
|
|
|
|
|
Scala tympani |
GFP |
|
|
|
|
|
|
|
|
|
|
|
|
Rat |
Rupture of |
Spiral ligament, |
AAV.CMV-XIAP |
1012 pfu, |
14 days |
Hair cell |
Cooper et al. |
|
RWM |
organ of Corti, |
|
2 or 5 μl |
|
protection |
[61] |
|
|
spiral neurons |
|
|
|
from |
|
|
|
|
|
|
|
cisplatin |
|
|
|
|
|
|
|
treatment |
|
|
|
|
|
|
|
|
|
26 |
Maeda · Sheffield · Smith |

Table 2. Continued
Animal |
Delivery route |
Expression in |
Vector |
Dose |
Duration |
Others |
Reference |
|
|
cochlea |
|
|
|
|
|
|
|
|
|
|
|
|
|
Guinea pig |
Cochleostomy |
Supporting cells |
Ad CMV– Math1, |
109 pfu, 2 μl |
30 days |
Vestibular |
Staecker et al. |
|
|
|
E1, E3, E4 deleted |
|
|
hair cell |
[59] |
|
|
|
|
|
|
regeneration |
|
|
|
|
|
|
|
|
|
Guinea pig |
Endolymphatic |
Stria vascularis, |
Sendai virus- |
5×107 pfu, |
3 days |
|
Kanzaki et al. |
|
perfusion |
spiral ligament, |
eGFP |
5 μl |
|
|
[69] |
|
|
organ of Corti |
|
|
|
|
|
|
|
|
|
|
|
|
|
Mouse |
Cochleostomy |
Spiral ligament, |
AAV.CMV-eGFP |
3×1010, 5 μl |
14 days |
|
Lui et al. [62] |
|
|
spiral limbus, |
AAV.CAG-eGFP |
|
|
|
|
|
|
organ of Corti, |
AAV.Myo7A- |
|
|
|
|
|
|
Reissner’s |
eGFP |
|
|
|
|
|
|
membrane, spiral |
|
|
|
|
|
|
|
neurons, inner hair |
|
|
|
|
|
|
|
cells |
|
|
|
|
|
|
|
|
|
|
|
|
|
Rat |
Rupture of |
Spiral ligament, |
AAV.CAG-GDNF, |
5×1010, 5 μl |
30 days |
Hair cell |
Liu et al. [63] |
|
RWM |
spiral limbus, |
Tet-On |
|
|
protection |
|
|
|
organ of Corti, |
|
|
|
from |
|
|
|
Reissner’s |
|
|
|
kanamycin |
|
|
|
membrane, spiral |
|
|
|
treatment |
|
|
|
neurons |
|
|
|
|
|
|
|
|
|
|
|
|
|
Guinea pig |
Endolymphatic |
Supporting cells |
AAV5.CMV-eGFP |
3.7×109, |
30 days |
|
Ballana et al. |
|
perfusion |
|
|
100 μl |
|
|
[68] |
|
|
|
|
|
|
|
|
promoter. Direct infusion of LV vectors resulted in eGFP transgene expression only in the lining cells of the scala tympani [64].
Indirect Approaches to Perilymphatic Systems
A more atraumatic method was studied by Jero et al. [65] who demonstrated the feasibility of diffusion of liposome-complexed plasmid and AV vector through the intact RWM of mice. These investigators were able to observe eGFP and β-gal transgene expression in the spiral limbus, spiral ligament, sensory and supporting cells of the organ of Corti, Reissner’s membrane and spiral ganglion cells 3–7 days after placing gelfoam soaked with a lipocomplexed plasmid directly on the RWM [66].
We used a similar approach to introduce a cytomegalovirus (CMV)-driven, domi- nant-negative GJB2 mutant construct into the inner ear and also observed expression in the spiral limbus, spiral ligament, epithelial cells in the basilar membrane, outer hair cells, inner and outer pillar cells, and Claudius cells in the organ of Corti [2]. The hearing loss associated with the mutant transgene expression was significant at 1–3 days after transfection, but as expected not at 5 days after transfection (fig. 5).
RNA Interference in the Inner Ear |
27 |
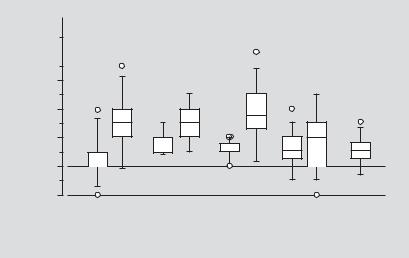
Intraaural difference of ABR threshold
50 |
* |
* |
* |
|
n.s. |
||||
40 |
|
|
|
|
|
|
|
|
|
|
|
|
|
|
|
|
|
|
30
20
10
0
–10
1 |
2 |
|
1 |
2 |
|
|
1 |
2 |
|
|
1 |
2 |
|
2 |
1 day after |
2 days after |
3 days after |
5 days after |
12 days after |
||||||||||
treatment |
|
treatment |
|
treatment |
|
treatment |
treatment |
Fig. 5. Hearing loss associated with dominant-negative GJB2 mutant expression in mice (n = 7–10
for each group). A plasmid vector expressing the dominant-negative GJB2R75W-eGFP (0 5 μg/μl) was complexed with DOTAP liposome, soaked in gel foam and applied to the intact round window mem-
brane (1–5 μl). The ABR threshold in the treated ear is expressed as the intra-aural difference from the untreated ear to minimize variation between animals. Transient hearing loss was induced in the plas- mid-applied ear 1, 2 and 3 days after treatment but hearing levels were nearly normal by 5 days. After 12 days, hearing levels were equivalent in both treated and control animals. Whiskers = 1.5 SD from the median; = outliers >1.5 SD from the median. 1 = DOTAP/cholesterol only; 2 = lipocomplexed pGJB2R75W-eGFP (0.25 μg/μl). Mann-Whitney U test: *p < 0.01; n.s. = not significant.
Direct Approaches to Endolymphatic System
Surgical approaches to the endolymphatic sac allow drug delivery to the endolymphatic system, resulting primarily in transgene expression in the endolymphatic duct epithelium and transitional epithelium in the utricle and saccule. Yamasoba et al. [67] reported that after high-dose injection of Ad vector (1 × 1010 particles/ml, 10–15 μl), LacZ expression could be detected in cells bordering the scala media, including endothelial cells in Reissner’s membrane, marginal cells in the stria vascularis and supporting cells in the organ of Corti. Endolymphatic perfusion with an AAV5 vector produced expression limited to supporting cells [68], while expression in the spiral ligament, organ of Corti and spiral ganglion was noted after endolymph injection of a Sendai virus vector [69].
Advantages and Disadvantages of These Approaches
There are many potential complications associated with gene delivery into the inner ear regardless of the route of delivery, including iatrogenic cell damage from hydropressure and ototoxicity of the delivery vehicle. In rodent experiments, after cochleostomy and injection to scala tympani, damage of inner ear cells and an inflammatory
28 |
Maeda · Sheffield · Smith |
response are occasionally seen [70], although it is relatively uncommon to observe inner ear cytoarchitectural damage and inflammation at the light microscopic levels. The damage that is observed is usually confined to the basal turn of the cochlea or the site of injection at the RWM.
In experiments in which Ad vectors have been perfused into the scala tympani by osmotic pumps, replication-deficient Ad (E1–, E3–) vectors presumably affected the function of transfected outer hair cells as evidenced by compromised distortion product otoacoustic emissions, while replication defective Ad (E1–, E3–, pol–) vectors did not [71]. After cochleostomy and infusion of AAV [59], LV vectors [63], or liposome vectors [72], the cytoarchitecture of the cochlea was intact and free from inflammation, with the exception of mild fibrosis at the injection site. Derby et al. [73] observed a lymphocytic infiltration in the cochlea after injection of HSV vector or vaccinia virus vectors into scala tympani, highlighting the importance of transgene selection in circumventing complications like loss of cell viability and inflammation.
The duration of transgene expression is also an important factor that must be studied under comparative experimental conditions. Using direct injection of Ad vectors driven by CMV or Rous sarcoma virus promoters, transgene expression in the cochleae was detected 28 and 56 days after transfection in mice and guinea pigs, respectively [58, 74]. AAV, HSV and LV vectors can potentially offer permanent transgene expression by chromosomal integration, which would be beneficial for ‘one-time’ therapies to correct hereditary deafness. These vectors, however, show a more limited cell-type tropism in the inner ear when compared to Ad vectors, with most published data using direct injection of Ad into the perilymphatic system of rodents showing substantial transduction of sensory and supporting cells in the organ of Corti.
Clearly, an indirect approach through the intact or chemically permeabilized RWM reduces iatrogenic damage to a minimum, however transgene expression is relatively low and primarily in the basal turn of the cochlea. The RWMs of various species differ in their thickness (70 μm in humans, 40–60 μm in rhesus monkeys, 10–14 μm in chinchillas) but share a similar composition consisting of outer and inner epithelial layers between which lies a connective tissue core. Passage of substances from the middle to the inner ear via the RWM is an active process by the epithelia as demonstrated with cationic ferritin, horseradish peroxidase and 1-μm latex spheres in experiments using rodents and monkeys [75]. In studies of guinea pigs, Suzuki et al. [76] were able to enhance permeability of the outer epithelium of the RWM by phenol-containing local anesthetics. Application of Ad vector into the middle ear of these animals was followed by transgene expression in the sensory epithelium of the cochlea [76].
Vectors to Deliver siRNAs in Vivo
In rodent experiments, in vivo transfection of siRNA has been achieved using a number of different vectors in a number of different organs. Surprisingly, even naked
RNA Interference in the Inner Ear |
29 |
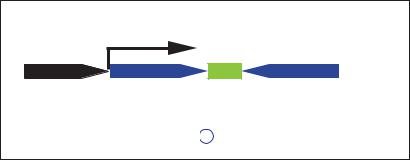
Small hairpin RNA expression limit
Transcription
5’
Pol III promoter |
|
|
|
|
|
|
Target sequence |
Spacer |
|||||||||||||||||||||
|
|
|
|
|
|
|
|
|
|
|
Sense |
|
|||||||||||||||||
Small hairpin RNA |
|
|
|
|
|
|
|
|
|
|
|
|
|
|
|
|
|
|
|
|
|
|
|
|
|
|
|
|
|
3’ |
5’ |
|
|
|
|
|
|
|
|
|
|
|
|
|
|
|
|
|
|
|
|
|
|
|
|
|
|||
|
|
|
|
|
|
|
|
|
|
|
|
|
|
|
|
|
|
|
|
|
|
|
|
|
|
|
|
|
|
|
|
|
|
|
|
|
|
|
|
|
|
|
|
|
|
|
|
|
|
|
|
|
|
|
|
|
|
|
Targetsequence |
TTTTT3’ |
Antisense Transcription termination signal
Fig. 6. Scheme of RNA polymerase III (Pol III) driven, small hairpin RNA (shRNA) expression cassette. Pol III promoter (H1 or U6 promoter) is followed by DNA template that corresponds to the target sequence of the gene to be suppressed. The motif of inverted repeats is separated by a short spacer sequence and followed by transcriptional termination signal of five thymidines. The transcript folds back on itself to form shRNA.
synthetic siRNA has been successfully introduced and has functioned in the cells of organs such as the liver and kidney following hydrodynamic injection in mouse tail veins. However, naked synthetic RNA is degraded in serum unless it is modified for stabilization making this transfection route less than ideal for clinical use. In spite of this, a recent study demonstrated suppression of gene expression in peripheral blood leukocytes following intravenous administration of synthetic siRNA [77]. Intratumoral injection of naked synthetic siRNA has been used to suppress K-Ras in cancer cells, making chemotherapy more effective [78], while intradermal siRNA injection suppressed the expression of a mutated gene in skin [79]. Cationic liposomes, polyethylenimine vectors and atelocollagen are alternate efficient methods to deliver synthetic siRNAs by either topical or systemic injection [80]. If synthetic siRNAs are used, RNAi will remain a transient phenomenon, but in certain cases this may be acceptable, as in treatments to suppress pain [81].
To achieve long-term stable expression of siRNA, plasmid vectors with RNAi expression units driven by RNA Pol III promoters U6 and H1 have been developed by several researchers [82–85]. Pol III transcribes significantly shorter RNAs than those transcribed by RNA polymerases I and II (Pol II), with a short stretch of about 5 thymidine residues serving as the termination signal. The expression cassette contains an shRNA sequence of an inverted repeat of 19–29 nt separated by a short spacer sequence. The transcribed RNA molecule forms short hairpin RNA that is indistinguishable from siRNAs in terms of RNAi efficiency and mechanism (fig. 6).
By using a RNA Pol II promoter, Xia et al. [86] developed an shRNA-expression plasmid with a modified CMV promoter, a downstream shRNA template with inverted repeat and a minimal polyA cassette. In this expression unit, the hairpin sequence was placed immediately adjacent to the transcription initiation site of the promoter [86].
30 |
Maeda · Sheffield · Smith |
Ad and AAV vectors with a Pol II or modified CMV shRNA expression unit have been demonstrated to induce in vivo RNAi in neural tissues. In one study, an Ad vector with a modified CMV expression cassette of shRNA against eGFP was directly injected to the basal ganglia of transgenic mice expressing eGFP. Western blot analysis showed that eGFP expression was diminished in the injected hemisphere 5 days after injection [86]. The same group also injected an AAV vector with the H1-shRNA expression cassette into the cerebellum of a transgenic murine model of spinocerebellar ataxia type 1. Expression of shRNA targeting human ataxin1 was verified 10 days after the injection [51]. When they injected AAV.U6-shRNA vectors into the basal ganglia of a murine model of Huntington’s disease, shRNA expression against the human Huntington’s disease gene was detected 21 days after injection [52]. In these experiments using AAV vectors, a CMV-driven, eGFP expression unit was included in the chimeric viral constructs, and eGFP expression was detected in the injected sites up to 5 months later.
Lentiviral vectors with H1-shRNA expression units have been used to treat a mouse model of amyotrophic lateral sclerosis (ALS) that expresses a human SOD1 mutation. The SOD1 mutant causes a dominantly inherited form of ALS through a gain-of-function mechanism, and the lentiviral-mediated shRNA selectively targets the expression of the mutant gene. In two studies using these animals, lentiviral vectors were injected intraspinally [87] or intramuscularly (utilizing retrograde axonal transport to motoneurons) [88]. Western blotting and immunofluorescence demonstrated a reduction in SOD1 mutant protein expression 15–50 days after spinal injection and an improvement was observed in motor neuron survival and motor ability 80–100 days after the spinal and intramuscular injection.
Conclusions
The development of technologies to induce specific gene suppression by small DNA or RNA molecules is outpacing the development of appropriate drug delivery systems that are applicable to the inner ear. The Ad vector with poi II or modified CMV promoters that expresses shRNA has the potential to induce RNAi in the inner ear for relatively short periods of time (up to 2 months).
Future studies focusing on methods for efficient and substantial long-term transfection into the inner ear sensory epithelium are crucial if RNAi-based gene therapy of hereditary deafness caused by gain-of-function mechanisms is to become a clinical reality.
Acknowledgments
This research was supported by NIH grant DC03544 (R.J.H.S.).
RNA Interference in the Inner Ear |
31 |
References
1 Van De Water TR, Lallemend F, Eshraghi AA, Ahsan S, He J, Guzman J, Polak M, Malgrange B, Lefebvre PP, Staecker H, Balkany TJ: Caspases, the enemy within, and their role in oxidative stressinduced apoptosis of inner ear sensory cells. Otol Neurotol 2004;25:627–632.
2 Maeda Y, Fukushima K, Nishizaki K, Smith RJ: In vitro and in vivo suppression of GJB2 expression by RNA interference. Hum Mol Genet 2005;14:1641– 1650.
3 Wilkie AO: The molecular basis of genetic dominance. J Med Genet 1994;31:89–98.
4 Denoyelle F, Lina-Granade G, Plauchu H, Bruzzone R, Chaïb H, Lévi-Acobas F, Weil D, Petit C: Connexin 26 gene linked to a dominant deafness. Nature 1998;393:319–320.
5 Rouan F, White TW, Brown N, Taylor AM, Lucke TW, Paul DL, Munro CS, Uitto J, Hodgins MB, Richard G: trans-dominant inhibition of connexin43 by mutant connexin-26:implications for dominant connexin disorders affecting epidermal differentiation. J Cell Sci 2001;114:2105–2113.
6 Richard G, White TW, Smith LE, Bailey RA, Compton JG, Paul DL, Bale SJ: Functional defects of Cx26 resulting from a heterozygous missense mutation in a family with dominant deaf-mutism and palmoplantar keratoderma. Hum Genet 1998;103: 393–399.
7 Marziano NK, Casalotti SO, Portelli AE, Becker DL, Forge A: Mutations in the gene for connexin 26 (GJB2) that cause hearing loss have a dominant negative effect on connexin 30. Hum Mol Genet 2003;12:805–812.
8 Kubisch C, Schroeder BC, Friedrich T, Lütjohann B, El-Amraoui A, Marlin S, Petit C, Jentsch TJ: KCNQ4, a novel potassium channel expressed in sensory outer hair cells, is mutated in dominant deafness. Cell 1999;96:437–446.
9 Coucke PJ, Van Hauwe P, Kelley PM, Kunst H, Schatteman I, Van Velzen D, Meyers J, Ensink RJ, Verstreken M, Declau F, Marres H, Kastury K, Bhasin S, McGuirt WT, Smith RJ, Cremers CW, Van de Heyning P, Willems PJ, Smith SD, Van Camp G: Mutations in the KCNQ4 gene are responsible for autosomal dominant deafness in four DFNA2 families. Hum Mol Genet 1999;8:1321–1328.
10 Kharkovets T, Hardelin JP, Safieddine S, Schweizer M, El-Amraoui A, Petit C, Jentsch TJ: KCNQ4, a K+ channel mutated in a form of dominant deafness, is expressed in the inner ear and the central auditory pathway. Proc Natl Acad Sci USA 2000;97:4333– 4338.
11 Van Laer L, Huizing EH, Verstreken M, van Zuijlen D, Wauters JG, Bossuyt PJ, Van de Heyning P, McGuirt WT, Smith RJ, Willems PJ, Legan PK, Richardson GP, Van Camp G: Nonsyndromic hearing impairment is associated with a mutation in DFNA5. Nat Genet 1998;20:194–197.
12 Yu C, Meng X, Zhang S, Zhao G, Hu L, Kong X: A 3-nucleotide deletion in the polypyrimidine tract of intron 7 of the DFNA5 gene causes nonsyndromic hearing impairment in a Chinese family. Genomics 2003;82:575–579.
13 Bischoff AM, Luijendijk MW, Huygen PL, van Duijnhoven G, De Leenheer EM, Oudesluijs GG, Van Laer L, Cremers FP, Cremers CW, Kremer H: A novel mutation identified in the DFNA5 gene in a Dutch family: a clinical and genetic evaluation. Audiol Neurootol 2004;9:34–46.
14 Gregan J, Van Laer L, Lieto LD, Van Camp G, Kearsey SE: A yeast model for the study of human DFNA5, a gene mutated in nonsyndromic hearing impairment. Biochim Biophys Acta 2003;1638:179– 186.
15 Van Laer L, Vrijens K, Thys S, Van Tendeloo VF, Smith RJ, Van Bockstaele DR, Timmermans JP, Van Camp G: DFNA5: hearing impairment exon instead of hearing impairment gene? J Med Genet 2004;41: 401–406.
16 Zhao YZ, Luo YK, Liang HD, Mei XG, Tang J, Lu CT, Zhang Y, Lin Q: Comparing transfection efficiency and safety for antisense oligodeoxyribonucleotide between phospholipids-based microbubbles and liposomes. J Drug Target 2006;14:687–693.
17 Juliano RL, Dixit VR, Kang H, Kim TY, Miyamoto Y, Xu D: Epigenetic manipulation of gene expression: a toolkit for cell biologists. J Cell Biol 2005;169: 847–857.
18 Zamaratski E, Pradeepkumar PI, Chattopadhyaya J: A critical survey of the structure-function of the antisense oligo/RNA heteroduplex as substrate for RNase H. J Biochem Biophys Methods 2001;48:189– 208.
19 Kurreck J: Antisense technologies. Improvement through novel chemical modifications. Eur J Biochem 2003;270:1628–1644.
20 Gleave ME, Monia BP: Antisense therapy for cancer. Nat Rev Cancer 2005;5:468–479.
21 Marwick C: First ‘antisense’ drug will treat CMV retinitis. JAMA 1998;280:871.
22 Dove A: Isis and antisense face crucial test without Novartis. Nat Biotechnol 2000;18:19.
32 |
Maeda · Sheffield · Smith |
23 Sandrasagra A, Leonard SA, Tang L, Teng K, Li Y, Ball HA, Mannion JC, Nyce JW: Discovery and development of respirable antisense therapeutics for asthma. Antisense Nucleic Acid Drug Dev 2002;12: 177–181.
24 Delprat B, Boulanger A, Wang J, Beaudoin V, Guitton MJ, Ventéo S, Dechesne CJ, Pujol R, Lavigne-Rebillard M, Puel JL, Hamel CP: Downregulation of otospiralin, a novel inner ear protein, causes hair cell degeneration and deafness. J Neurosci 2002;22:1718–1725.
25 Morishita R, Gibbons GH, Horiuchi M, Nakajima M, Ellison KE, Lee W, Kaneda Y, Ogihara T, Dzau VJ: molecular delivery system for antisense oligonucleotides: enhanced effectiveness of antisense oligonucleotides by HVJ-liposome mediated transfer. J Cardiovasc Pharmacol Ther 1997;2:213–222.
26 Morishita R, Gibbons GH, Horiuchi M, Ellison KE, Nakama M, Zhang L, Kaneda Y, Ogihara T, Dzau VJ: A gene therapy strategy using a transcription factor decoy of the E2F binding site inhibits smooth muscle proliferation in vivo. Proc Natl Acad Sci USA 1995;92:5855–5859.
27 Morishita R, Sugimoto T, Aoki M, Kida I, Tomita N, Moriguchi A, Maeda K, Sawa Y, Kaneda Y, Higaki J, Ogihara T: In vivo transfection of cis element ‘decoy’ against nuclear factor-kappaB binding site prevents myocardial infarction. Nat Med 1997;3:894–899.
28 Morishita R, Aoki M, Ogihara T: Does gene therapy become pharmacotherapy? Exp Physiol 2005;90: 307–313.
29 Watanabe K, Inai S, Jinnouchi K, Bada S, Hess A, Michel O, Yagi T: Nuclear-factor kappa B (NF-kappa B)-inducible nitric oxide synthase (iNOS/NOS II) pathway damages the stria vascularis in cisplatintreated mice. Anticancer Res 2002;22:4081–4085.
30 Jiang H, Sha SH, Schacht J: NF-kappaB pathway protects cochlear hair cells from aminoglycosideinduced ototoxicity. J Neurosci Res 2005;79:644– 651.
31Nagy I, Monge A, Albinger-Hegyi A, Schmid S, Bodmer D: NF-kappaB is required for survival of immature auditory hair cells in vitro. J Assoc Res
Otolaryngol 2005;1–9.
32 Kore AR, Vaish NK, Kutzke U, Eckstein F: Sequence specificity of the hammerhead ribozyme revisited; the NHH rule. Nucleic Acids Res 1998;26:4116– 4120.
33 Citti L, Rainaldi G: Synthetic hammerhead ribozymes as therapeutic tools to control disease genes. Curr Gene Ther 2005;5:11–24.
RNA Interference in the Inner Ear
34 Davies G, Martin TA, Ye L, Lewis-Russell JM, Mason MD, Jiang WG: Phospholipase-C gamma-1 (PLCgamma-1) is critical in hepatocyte growth factor induced in vitro invasion and migration without affecting the growth of prostate cancer cells. Urol Oncol 2008;26:386–391.
35 Michienzi A, Rossi JJ: Intracellular applications of ribozymes. Methods Enzymol 2001;341:581–596.
36 Beigelman L, McSwiggen JA, Draper KG, Gonzalez C, Jensen K, Karpeisky AM, Modak AS, MatulicAdamic J, DiRenzo AB, Haeberli P, Sweedler D, Tracz D, Grimm S, Wincott FE, Thackray VG, Usman N: Chemical modification of hammerhead ribozymes. Catalytic activity and nuclease resistance. J Biol Chem 1995;270:25702–25708.
37 Usman N, Blatt LM: Nuclease-resistant synthetic ribozymes: developing a new class of therapeutics. J Clin Invest 2000;106:1197–1202.
38 Zinnen SP, Domenico K, Wilson M, Dickinson BA, Beaudry A, Mokler V, Daniher AT, Burgin A, Beigelman L: Selection, design, and characterization of a new potentially therapeutic ribozyme. RNA 2002;8:214–228.
39 Ciafre SA, Niola F, Wannenes F, Farace MG: An anti-VEGF ribozyme embedded within the adenoviral VAI sequence inhibits glioblastoma cell angiogenic potential in vitro. J Vasc Res 2004;41: 220–228.
40 Wright L, Kearney P: Current status of ribozymes as gene therapy agents for cancer. Cancer Invest 2001;19:495–509.
41 Suzuki T, Anderegg B, Ohkawa T, Irie A, Engebraaten O, Halks-Miller M, Holm PS, Curiel DT, KashaniSabet M, Scanlon KJ: Adenovirus-mediated ribozyme targeting of HER-2/neu inhibits in vivo growth of breast cancer cells. Gene Ther 2000;7:241– 248.
42 Tomari Y, Zamore PD: Perspective: machines for RNAi. Genes Dev 2005;19:517–529.
43 Bertrand JR, Pottier M, Vekris A, Opolon P, Maksimenko A, Malvy C: Comparison of antisense oligonucleotides and siRNAs in cell culture and in vivo. Biochem Biophys Res Commun 2002;296: 1000–1004.
44 Vickers TA, Koo S, Bennett CF, Crooke ST, Dean NM, Baker BF: Efficient reduction of target RNAs by small interfering RNA and RNase H-dependent antisense agents. A comparative analysis. J Biol Chem 2003;278:7108–7118.
45 Elbashir SM, Martinez J, Patkaniowska A, Lendeckel W, Tuschl T: Functional anatomy of siRNAs for mediating efficient RNAi in Drosophila melanogaster embryo lysate. EMBO J 2001;20:6877–6888.
33