
Diss / 10
.pdfPrinciples of Systems Approach to Design Complex Radar Systems |
23 |
REFERENCES
1.Skolnik, M.I. 2008. Radar Handbook. 3rd edn. New York: McGraw-Hill, Inc.
2.Tzvetkov, A. 1971. Principles of Quantitative Ratings of Complex Radar System Efficiency. Moscow, Russia: Soviet Radio.
3.Hovanessian, S. 1984. Radar System Design and Analysis. Norwood, MA: Artech House, Inc.
4.Meyer, D. and H. Mayer. 1973. Radar Target Detection: Handbook of Theory and Practice. New York: Academic Press.
5.Drujinin, B. and D. Kontorov. 1976. Problems of Military Systems Engineering. Moscow, Russia: Military Press.
6.Gutkin, L.S., Pestryakov, V.V., and V.H. Tipugin. 1970. Radio Control. Moscow, Russia: Soviet Radio.
7.Skolnik, M.I. 2001. Introduction to Radar Systems. New York: McGraw-Hill, Inc.
8.Lacomme, P., Hardange, J.-P., Marchais, J.-C., and E. Normant. 2001. Air and Spaceborne Radar Systems: An Introduction. New York: William Andrew Publishing.
9.Tait, P. 2005. Introduction to Radar Target Recognition. Cornwall, U.K.: IEE Press.
10.Levanon, N. and E. Mozeson. 2004. Radar Signals. New York: IEEE Press, John Wiley & Sons, Inc.
11.Barton, D.K. 2005. Modern Radar System Analysis and Modeling. Canton, MA: Artech House, Inc.
12.Stimson, G.W. 1998. Introduction to Airborne Radar. 2nd edn. Raleigh, NC: SciTech Publishing, Inc.
13.Nitzberg, R. 1999. Radar Signal Processing and Adaptive Systems. Norwood, MA: Artech House, Inc.
14.Streetly, M. 2000. Radar and Electronic Warfare Systems. 11th edn. Surrey, U.K.: James Information Group.
15.Guerci, J.R. 2003. Space-Time Adaptive Processing for Radar. Norwood, MA: Artech House, Inc.
16.Barkat, M. 2005. Signal Detection and Estimation. 2nd Edn., Norwood, MA: Artech House, Inc.
17.DiFranco, J.V. and W.L. Rubin. 1980. Radar Detection. Norwood, MA: Artech House, Inc.
18.Edde, B. 1993. Radar Principles, Technology, Application. Englewood Cliffs, NJ: Prentice Hall, Inc.
19.Kay, S.M. 1993. Fundamentals of Statistical Signal Processing—Estimation Theory. Vol. I. Englewood Cliffs, NJ: Prentice Hall, Inc.
20.Kay, S.M. 1998. Fundamentals of Statistical Signal Processing—Detection Theory. Vol. II. Englewood Cliffs, NJ: Prentice Hall, Inc.
21.Knott, E.F., Shaeffer, J.F., and M.T. Tuley. 1993. Radar Cross Section. 2nd edn. Norwood, MA: Artech House, Inc.
22.Mahafza, B.R. 1998. Introduction to Radar Analysis. Boca Raton, FL: CRC Press, Taylor & Francis Group.
23.Mahafza, B.R. 2000. Radar System Analysis and Design Using MATLAB. Boca Raton, FL: CRC Press.
24.Nathanson, F.E. 1991. Radar Design Principles. 3rd edn. New York: McGraw Hill, Inc.
25.Peebles, Jr., P.Z. 1998. Radar Principles. New York: John Wiley & Sons, Inc.
26.Richards, M.A. 2005. Fundamentals of Radar Signal Processing. Englewood Cliffs, NJ: Prentice Hall, Inc.
27.Brookner, E. 1988. Aspects of Modern Radar. Boston, MA: Artech House, Inc.
28.Franceschetti, G. and R. Lanari. 1999. Synthetic Aperture Radar Processing. Boca Raton, FL: CRC Press.
29.Johnson, D.H. and D.E. Dudgeon. 1993. Array Signal Processing: Concepts and Techniques. Englewood Cliffs, NJ: Prentice Hall, Inc.
30.Klemm, R. 1998. Space-Time Adaptive Signal Processing: Principles and Applications. London, U.K.: INSPEC/IEEE.
31.Sullivan, R.J. 2000. Microwave Radar: Imaging and Advanced Concepts. Boston, MA: Artech House, Inc.
32.Tuzlukov, V.P. 2001. Signal Detection Theory. New York: Springer-Verlag.
33.Tuzlukov, V.P. 2002. Signal Processing Noise. Boca Raton, FL: CRC Press.
34.Tuzlukov, V.P. 2004. Signal and Image Processing in Navigational Systems. Boca Raton, FL: CRC Press.
35.Van Trees, H.L. 2002. Optimum Array Processing: Part IV of Detection, Estimation, and Modulation Theory. New York: John Wiley & Sons, Inc.
36.Tsui, J.B. 2004. Digital Techniques for Wideband Receivers. 2nd edn. Raleigh, NC: SciTech Publishing, Inc.
37.Bogler, P.L. 1990. Radar Principles with Applications to Tracking Systems. New York: John Wiley & Sons, Inc.
38.Cumming, I.G. and F.N. Wong. 2005. Digital Signal Processing of Synthetic Aperture Radar Data. Norwood, MA: Artech House, Inc.
39.Long, M.W. 2001. Radar Reflectivity of Land and Sea. 3rd edn. Boston, MA: Artech House, Inc.

2 Signal Processing by Digital Generalized Detector
in Complex Radar Systems
2.1 ANALOG-TO-DIGITAL SIGNAL CONVERSION: MAIN PRINCIPLES
For digital signal processing of target return signals in complex radar systems (CRSs), there is a need to carry out an analog-to-digital signal conversion. This transformation is produced by two stages. In the course of the first stage, there is a need to sample the target return signal—the continuous target return signal x(t) is sampled instantaneously and at a uniform rate, once every Ts seconds. In the course of the second stage—quantization—the sampled target return signal {x(nTs)} is converted into a sequence of binary coded words. The sampling and quantization functions are realized by analog-to-digital converters.
The design process of analog-to-digital converters involves particularly the task of choosing a sampling interval value and number of signal quantization levels of the sampled target return signal. Simultaneously, we should take into account the problems of designing and realization both for converters and for digital receivers processing the target return signals. In the present section, we consider and discuss the main aspects and foundations of analog-to-digital signal conversion and the main principles underlying the design of analog-to-digital converters.
2.1.1 Sampling Process
In general, a sampling process of the continuous function x(t) representing a target return signal is to measure its values at the time instants spaced Ts seconds apart. Consequently, we obtain an infinite sequence of samples spaced Ts seconds apart and denoted by {x(nTs)}, where n takes on all possible integer values, both positive and negative. We refer to Ts as the sampling period or sampling interval, and to its reciprocal, fs = 1/Ts, as the sampling rate. This ideal form of sampling is called an instantaneous sampling. As a rule, a value of Ts is constant.
A sampling device can be considered as a make circuit with the time τ and sampling period Ts. Time diagram of conversion of the continuous function x(t) into a sequence of instantaneous (τ → 0) readings {x(nTs)} spaced Ts seconds apart is shown in Figure 2.1. Let xδ(t) denote the signal obtained by individually weighting the elements of a periodic sequence of Dirac delta functions spaced Ts seconds apart by the sequence of numbers {x(nTs)} [1]:
∞ |
|
xδ (t) = ∑ x(nTs )δ(t − nTs ). |
(2.1) |
n= −∞
We refer to xδ(t) as the instantaneously (ideal) sampled target return signal. The term δ(t − nTs) represents a delta function positioned at time t = nTs. From the definition of the delta function [2], recall that such an idealized function has unit area. We may, therefore, view the multiplying factor x(nTs) in (2.1) as a “mass” assigned to the delta function δ(t − nTs). A delta function weighted in this manner is closely approximated by a rectangular pulse of duration τ and amplitude x(nTs)/τ; the smaller we make τ, the better the approximation will be.
25
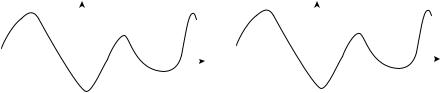
26 |
|
|
|
|
|
|
|
Signal Processing in Radar Systems |
|||||||||||||
|
|
|
x(t) |
|
|
|
|
|
|
|
xδ(t) |
||||||||||
|
|
|
|
|
|
|
|
|
|
||||||||||||
|
|
|
|
|
|
|
|
|
|
|
|
|
|
|
|
|
|
|
|
|
t |
|
|
|
|
|
|
|
|
|
|
|
|
|
|
|
|
|
|
|
|
|
|
|
|
|
|
|
|
|
|
|
|
|
|
|
|
|
|
|
|
|
|
|
|
|
|
|
|
|
|
|
|
|
|
|
|
|
|
|
|
|
|
|
|
|
|
|
|
|
|
|
|
|
|
|
|
|
|
|
|
|
|
|
|
|
|
|
|
|
|
|
|
|
|
|
|
|
|
|
|
|
|
|
|
|
|
|
|
|
|
|
|
|
|
|
|
|
|
|
|
|
|
|
|
|
|
|
|
|
|
|
|
|
|
|
t |
Ts |
|||||||||||||||||
|
|
|
|
|
|
|
|
|
|
|
|
|
|
|
|
|
|
||||
|
|
|
|
|
|
|
|
|
|
|
|
|
|
|
|
|
|
|
|
||
(a) |
|
|
|
(b) |
|
|
|
|
|
|
|
|
|
|
|
||||||
|
|
|
|
|
|
|
|
|
|
|
|
|
|
||||||||
|
|
|
|
FIGURE 2.1 Illustration of the sampling process: (a) Analog waveform x(t) and (b) instantaneously sampled representation of x(t).
The instantaneously sampled target return signal xδ(t) has a mathematical form similar to that of the Fourier transform of a periodic signal. This is readily established by comparing (2.1) for xδ(t) with the Fourier transform of a periodic signal given by
∞ |
∞ |
|
∑ x(t − mTs ) ↔ fs ∑G(nfs )δ( f − nfs ), |
(2.2) |
|
m= −∞ |
n= −∞ |
|
where G(nfs) is the Fourier transform of x(t), evaluated at the frequency f = nfs. This correspondence suggests that we may determine the Fourier transform of the sampled target return signal xδ(t) by invoking the duality property of the Fourier transform [3], the essence of which is the following:
If x(t) ↔ G( f ), then G(t) ↔ g(− f ). |
(2.3) |
Representation of the continuous function x(t) in the form of the sequence {x(nTs)} is possible only under the well-known restrictions. One restriction in kind is a requirement of spectrum limitation of the sampled target return signal xδ(t). By Kotelnikov’s theorem concerning the signal space concept [4], the continuous target return signal x(t) possessing a limited spectrum is completely defined by countable set of samples spaced Ts ≤ 1/(2fmax) seconds apart, where fmax is the cutoff frequency of target return signal spectrum.
Under digital signal processing in CRSs, a stochastic process at the analog receiver output is an object to sample. This output process is a narrowband process at the condition fs / fc 1, where fs is the signal spectrum bandwidth and fc is the carrier frequency. This condition allows us to apply
an envelope procedure to represent the narrowband signal in the following form:
x(t) = X(t) cos[2πfct + ϕ(t)], |
(2.4) |
where
X(t) is the low-frequency signal (envelope)
φ(t) is the phase modulation law of the narrowband signal—slowly varied function in comparison with 2πfct
Since information about the target is extracted from the envelope X(t) and phase φ(t) of the target return signal and not from the carrier frequency fc, and, moreover, X(t) and φ(t) are the slowly varied functions in time, there is a need to convert the target return signal given by (2.4) in such a way that sampling intervals would be defined by the real signal spectrum bandwidth rather then the carrier frequency fc. In the case of the narrowband radio signal, we can write
|
|
|
, |
(2.5) |
x(t) = Re X(t)exp( j2πfct) |
||||
|
|
|
|
|

Signal Processing by Digital Generalized Detector in Complex Radar Systems |
27 |
where Re[·] is the real part of the complex narrowband signal and |
|
|
(2.6) |
X(t) = X(t) exp[ jϕ(t)] |
is the complex envelope of the narrowband signal. This complex envelope of the narrowband signal can be also presented in the following form:
|
(2.7) |
X(t) = X(t) cos ϕ(t) − jX(t)sin ϕ(t) = xI (t) − jxQ (t), |
where xI(t) and xQ(t) are, respectively, the in-phase and quadrature components of the narrowband target return signal x(t). Moreover,
X(t) = xI2 (t) + xQ2 (t), X(t) > 0, |
(2.8) |
|||
ϕ(t) = arctg |
xI (t) |
, −π ≤ ϕ(t) ≤ π. |
(2.9) |
|
xQ (t) |
||||
|
|
|
The in-phase xI(t) and quadrature xQ(t) components can be obtained by product between the target return signal x(t) and two orthogonal signals with the frequency fc forming at the local oscillator output. The corresponding device is called the phase detector. The flowchart of a simple phase detector is presented in Figure 2.2, where there are multipliers followed by the low-pass filters suppressing all high-frequency harmonics. Thus, the low-pass filters pass only the low-frequency in-phase xI(t) and xQ(t) quadrature components that must be sampled by the analog-to-digital converter.
The complex envelope of the narrowband radio signal can be presented either by the envelope and phase, which are the functions of time, or by the in-phase and quadrature components. In accordance with this statement, under the narrowband radio signal sampling there is a need to use two samples: either the envelope amplitude and phase or the in-phase and quadrature components of complex amplitude of the narrowband radio signal. Thus, we deal with two-dimensional signal sampling.
The sampling theorem for the two-dimensional signal may be presented in the following form [5]
Ts1 ≤ |
1 |
, Ts2 ≤ |
|
1 |
, |
(2.10) |
|
f |
f |
2max |
|||||
|
|
|
|
||||
|
1max |
|
|
|
|
where f1max and f2max are the highest frequencies in spectra of the first and second components of the narrowband signal. It is important to note that if we represent the narrowband signal using the envelope amplitude and phase, the frequencies then differ: namely, f1max is the maximal frequency of the amplitude-modulated narrowband signal spectrum; f2max is the maximal frequency of the
|
|
|
|
|
|
|
|
Low-pass |
|
|
ADC |
xI(mTs) |
|
|
|
|
|
|
|
|
|
|
|
|
|
||
|
|
|
|
|
|
|
|
filter |
|
|
|
|
|
|
|
|
|
|
|
|
|
|
|
|
|
|
|
x |
(t) |
|
|
|
cos2πfct |
|
|
|
xQ(mTs) |
||||
|
|
|
|
|
|
||||||||
|
|
|
|
|
|
|
|||||||
|
|
|
|
|
|
|
|
|
|
|
|
||
|
|
|
|
|
|
|
|
Low-pass |
|
|
ADC |
||
|
|
|
|
|
|
|
|
|
|
|
|
||
|
|
|
|
|
|
|
|
filter |
|
|
|
|
|
|
|
|
|
|
|
|
|
|
|
|
|
|
|
|
|
|
|
|
|
|
|
|
|
|
|
|
|
|
|
|
|
|
sin2πfct |
|
|
|
|
|
|||
|
|
|
|
|
|
|
|
|
|
||||
|
|
|
|
|
|
|
|
|
|
FIGURE 2.2 Example of phase detector: ADC—the analog-to-digital converter.

28 |
Signal Processing in Radar Systems |
phase-modulated narrowband signal spectrum. For example, in the case of a completely known deterministic signal, the spectral bandwidth of the envelope is defined from the following condition [6,7]:
f1max × τ0 = 1 or |
f1max = |
1 |
, |
(2.11) |
τ0 |
where τ0 is the duration of the sampled signal. Consequently, a maximal sampling period of amplitude envelope is limited by the condition Ts ≤ τ0. In doing so, an initial phase of the envelope is known. By this reason, it becomes evident that there is no need to sample it. In the case of a wideband signal of the same duration τ0 (the case of chirp modulation), the spectrum bandwidth of the modulated signal is close to double the amount of frequency deviation [8]:
f2max ≈ 2 F0. |
(2.12) |
Consequently, for one-valued representation of the frequency-modulated signal with constant amplitude, there is a need to set the phase
ϕ(t) = ∫ |
F0 dt |
(2.13) |
|||
at readings spaced as |
|
|
|
|
|
Tsϕ ≤ |
|
1 |
. |
(2.14) |
|
2 |
F0 |
||||
|
|
|
|||
Number of phase counts of the signal with duration τ0 is given by |
|
||||
Nϕ = 2 |
F0τ0. |
(2.15) |
Under the representation of complex envelope of the radio signal in the in-phase xI(t) and quadrature xQ(t) components, the maximal frequencies f1max and f2max are the same, that is,
f1max = f2max = fmax. |
(2.16) |
Consequently, the sampling must be carried out simultaneously over every equal sampling interval:
T |
≤ |
|
1 |
|
and |
T |
≤ |
|
1 |
. |
(2.17) |
|
|
|
|
|
|||||||
sI |
|
|
fmax |
|
sQ |
|
|
fmax |
|
||
|
|
|
|
|
|
|
|
||||
In the case of the signal with duration τ0 and random initial phase, we can write |
|
||||||||||
fmax = |
1 |
|
and |
TsI , TsQ |
≤ τ0. |
(2.18) |
|||||
|
|||||||||||
τ0 |
|
In the case of chirp-modulated signal with random initial phase, we have
fmax ≈ F0. |
(2.19) |

Signal Processing by Digital Generalized Detector in Complex Radar Systems |
29 |
Consequently, |
|
|
|
|
|
|
|
|
TsI |
, TsQ ≤ |
1 |
|
≤ |
|
1 |
|
(2.20) |
|
|
|
F0 |
|||||
|
|
fmax |
|
|||||
and the number of paired samples for the signal with duration τ0 is determined as |
|
|||||||
|
NI , NQ = |
F0τ0. |
|
|
(2.21) |
In the case of the phase-manipulated pulse signal, the number of paired samples must not be less than the number of elements in code chain. If τ0 is the duration of elementary signal, then the sampling intervals for the in-phase and quadrature components of the radio signal are given by
TsI , TsQ ≤ τ0. |
(2.22) |
2.1.2 Quantization and Signal Sampling Conversion
Under digital signal processing of target return signals in CRSs, there is a need to carry out a quantization of sampled values of complex envelope and phase or in-phase and quadrature components of radio signals in addition to sampling. Devices that carry out this function are called the quantizers.
The amplitude characteristic of alternating-sign sample quantizer with a fixed quantization step is presented in Figure 2.3. Here X1, X2,…, Xi, Xi+1,…, Xm are the decision values; X0 is the limiting
level of signal; x is the quantization step; and x1, x2 ,…, xm are the sampled data of output signal |
||||
covering the following center of range: |
′ ′ |
′ |
||
|
|
|
||
xi′ = |
Xi + Xi+1 |
. |
(2.23) |
|
2 |
||||
|
|
|
Under quantization of the in-phase and quadrature components of radio signal complex amplitude, the quantization step is chosen, as a rule, based on the condition
x = Xmin ≤ σ0 , |
(2.24) |
where σ20 is the variance of receiver noise.
|
|
x´ |
|
X0 |
|
|
|
x´m |
|
|
|
|
|
|
|
|
|
|
|
|
|
xi´+1 |
|
|
|
|
|
|
xi´ |
|
|
|
|
|
|
x2´ |
|
|
|
|
|
–Xi+1 –Xi |
–X2 –X1x´1 |
X1 |
X2 |
Xi |
Xi+1 Xm |
X |
|
|
–x1´ |
||||
|
|
–x´2 |
|
|
|
|
FIGURE 2.3 Alternating—sign sample quantizer with fixed quantization step.
30 Signal Processing in Radar Systems
The number of quantization levels is determined by
Nq = |
Xmax − Xmin |
= dr − 1, |
(2.25) |
|
|||
|
x |
|
where dr is the dynamic range of analog part of receiver. The number of bit site to represent the sampled target return signal is given by
nb = [log2 (Nq +1)] = [log2 dr ], |
(2.26) |
where [z] is the nearest integer NLE z. To characterize the analog-to-digital converter, we often use the following formula defining a dynamic range in dB for quantized sequence of samples for one bit of conversion [9]:
v = |
20 lg dr = |
20 lg dr |
≈ 6 dB . |
(2.27) |
|
[log2 dr ] |
|||||
|
nb |
bit |
|
Under detection of the target return signal, definition of its parameters, and noise compensation by digital signal processing subsystems in CRSs, there is a need to use a capacity digit, for example, Nb = 6 ÷ 8, analog-to-digital conversion of the sampled target return signal. Capacity digit quantization at a high sampling rate fs is a very difficult technical problem. Moreover, an increase in the sampling rate fs and capacity digit quantization leads to overdesign of digital signal processing subsystems in complex radar systems. Because of this, we use binary quantizers and binary detectors for target return signals, in addition to capacity digit quantization [10,11]. Binary detectors are very simple under realization by digital signal processing techniques.
2.1.3 Analog-to-Digital Conversion: Design Principles and Main Parameters
Manifold types of analog-to-digital conversion are employed by digital signal processing subsystems in CRSs, for instance, the analog-to-digital conversion of voltage/current, time intervals, phase, frequency, and angular displacement. The principal structure of majority of analog-to-digital converters is the same: There are sampling block, quantization block, and data encoding.
The main engineering data are as follows:
1.Time parameters defining a speed of operation (see Figure 2.4):
a.Sampling interval Ts
b.Time of conversion Tc, within the limits of which the target return signal is processed by the analog-to-digital converter
c.Time of conversion cycle Tcc, that is, the delay between the instant of appearance of the incoming target return signal at the input of the analog-to-digital converter and the instant of occurrence of output code generation
2.The number of code bits Nb of the target return signal
3.The element base of the analog-to-digital converter
Consider in detail the Q-factors or external parameters of analog-to-digital converter.
2.1.3.1 Sampling and Quantization Errors
There are two types of these errors: dynamical errors and statical errors. The dynamical errors are the discrete transform errors. The statical errors are the unit sample errors. The dynamical errors depend on the target return signal nature and time performance of the analog-to-digital

Signal Processing by Digital Generalized Detector in Complex Radar Systems |
31 |
X
Ts
t
Tc
x´
Tcc |
t
FIGURE 2.4 Time parameters of ADC.
converter. The main constituent of this kind of error is an inaccuracy caused by variations in the target return signal parameters at the analog-to-digital converter input. For example, if variations in the amplitude of the target return signal within the limits of Tcc are less or comparable with the quantization step x, then
Tcc ≤ |
x |
, |
(2.28) |
|
V |
||||
|
|
|
||
|
xmax |
|
|
where Vxmax is the maximal speed of variations of the sampled and quantized target return signal. Taking into consideration that Tccmax = Ts , we can define the sampling interval Ts based on (2.28).
The unit sample error is caused, first, by quantization error with variance
σq2 = |
x2 |
(2.29) |
|
12 |
|||
|
|
and, second, by deviation of actual quantization performance from ideal one (see Figure 2.3). If this deviation at the i-th quantization step is equal to ξi and the input target return signal possesses a uniform distribution of instantaneous values within the limits of operating range, then the variance of statical quantization error can be determined by
|
|
|
|
Nq |
|
|
|
σq2Σ = |
x2 |
+ |
1 |
∑ |
ξi2. |
(2.30) |
|
12 |
Nq |
||||||
|
|
|
|
||||
|
|
|
|
i=1 |
|
|
32 |
Signal Processing in Radar Systems |
2.1.3.2 Reliability
Reliability of the analog-to-digital converter is the ability to keep operation accuracy at given boundaries within the limits of a definite time interval under a given environment. As a rule, to evaluate the reliability of an analog-to-digital converter the probability of no-failure operation for time t is used, taking into account one of the following failure types:
•The outage—glitch
•Errors exceed a predefined value of accuracy—degradation failure
•Intermittent failure—malfunctions
An increase in the reliability of analog-to-digital converters is obtained owing to redundancy. Other parameters that pose a risk of limitation include power consumption, weight, and other
dimensions of analog-to-digital converters, cost under serial production, manufacturability, time that is required for design, and so on.
As a generalized Q-factor of analog-to-digital converter efficiency, we consider the following ratio:
= |
nb fs |
|
, |
(2.31) |
|||
Q |
|||||||
|
|
|
|
||||
where |
|
|
|
|
|
|
|
nb fs = |
nb |
|
|
(2.32) |
|||
Ts |
|
|
|||||
|
|
|
|
|
is the data throughput of the analog-to-digital converter and Q is the size of enginery. At the digital signal processing of wideband radio signals in CRSs, rigid requirements in data throughput and reliability are applied to analog-to-digital converters. To design and produce such converters, the parallel Fourier transforms for target return signals are used.
2.2 DIGITAL GENERALIZED DETECTOR FOR COHERENT IMPULSE SIGNALS
2.2.1 Matched Filter
Recall briefly the main statements of classical detection theory. In accordance with a general theory of radar signal processing, the signal processing in time of stochastic process x(t) representing an additive mixture of the signal s(t) and stationary white Gaussian noise w(t) is reduced to calculation of the correlation integral, which in the case of a scalar real signal s(t, α) with known parameter α can be presented in the following form:
T (α) = ∫∞ s (t, α)x(t)dt, |
(2.33) |
−∞ |
|
where s*(t, α) is the expected signal model generated by a local oscillator in the receiver or detector. If α is the delay of the expected signal with respect to the incoming initial input realization x(t), then the correlation integral is determined as
T (α) = ∫∞ s (t − α)x(t)dt. |
(2.34) |
−∞ |
|