
Biomedical EPR Part-B Methodology Instrumentation and Dynamics - Sandra R. Eaton
.pdfSATURATION RECOVERY EPR |
9 |
There is an inherent trade off between S/N and signal distortion. In practice, SR curves are initially recorded at higher observe power to facilitate signal detection, and then the observe power is decreased until no further increase in the recovery time constant can be detected. Yin and Hyde (1989) showed that the rates of bimolecular collisions between nitroxyl radicals can be measured in SR experiments that use observing power so high that the “effective is altered. The advantage of using higher observe power is higher signal-to-noise.
4.4Comparison of CW-Saturation Recovery with Inversion Recovery or Echo-Detected Saturation Recovery
Inversion recovery and echo-detected SR are two other pulse techniques that have been used to measure (Figure 1b,c). In an inversion recovery experiment the first pulse is a 180° pulse that inverts the spin magnetization. The time between the inverting pulse and the two-pulse spin echo sequence that is used to monitor the net magnetization is varied. The disadvantage of the inversion recovery sequence is that spectral diffusion processes with time constants that are shorter than
contribute to the recovery curve. In echo-detected SR (Figure 1c) a longer lower-power pulse is used to saturate the EPR transition and the net magnetization as a function of time is monitored with two-pulse spin echoes. In principle, the recovery curve obtained by echo-detected SR method should have the same time constant as obtained by CW-SR, if the lengths and powers of the saturating pulses are comparable.
An advantage of CW-SR is that the saturating microwave pulse is produced by a CW source, so it can, in principle, be made as long as is required to saturate the spectral diffusion processes. By contrast most sources used in spin echo and inversion recovery experiments are pulsed travelling wave tube (TWT) amplifiers for which the maximum length of a pulse is about The limitations on lengths of pulses can be partially overcome by using a series of pulses (a picket fence of pulses), but when this is done the length of time between the pulses in the pulse train must be short relative to the time constants for the spectral diffusion processes. Another advantage of CW detection is that it can be used even when
is so short, or echo envelope modulation is so deep, that it would be difficult to observe an echo. Short
can arise in fluid solutions of nitroxyl radicals where
is dominated by incomplete motional averaging of g and A anisotropy and
typically is substantially longer than
The relative benefits of long and short saturating pulses to obtain various kinds of relaxation information in CW-SR experiments have been described by Hyde and coworkers (Hyde,
10 |
SANDRA S. EATON AND GARETH R. EATON |
1979; Yin and Hyde, 1987b) and some examples are included in the following section.
Provided that is long enough to permit spin-echo detection, the echodetected SR frequently is more sensitive than CW-SR. The detection system in the CW-SR experiment does not use magnetic field modulation, because the modulation could function as a relaxation process or cause passage effects. Thus, an SR spectrometer does not benefit from the noise-rejection achieved by phase-sensitive detection at the modulation frequency that is used in most CW spectrometers. Also, it is necessary to use very low observe powers to avoid perturbing the system in a CW-SR experiment. However, the detector filter time constant, chosen appropriate to the signal recovery time constant, usually is much longer for CW detection than for echo detection. The longer filter time constant improves the signal-to-noise proportional to the square root of the time constant. An advantage of inversion recovery or echo-detected SR is that high power pulses are used to form the spin echoes. These pulses typically are “hard” pulses that fully invert the majority of the spins over a field range of several gauss. By contrast, in the CW-SR experiment the low observe power creates a “soft” pulse, which is only a small perturbation of the magnetization for the spins within a narrower field range. As a result, the CW-SR experiment detects a much smaller change in magnetization than the inversion recovery or spin echo experiment.
5.APPLICATIONS
The following paragraphs give brief descriptions of four of the more widely used applications of SR. The discussions are intended to show the range of information that can be obtained by SR and are not intended to be comprehensive reviews.
5.1Characterization of Electron Spin Relaxation Processes and Mechanisms
Values of measured by SR have been crucial in elucidating processes and mechanisms of electron spin relaxation. Many of these experiments and their interpretation are discussed in a chapter in a prior volume of this series (Eaton and Eaton, 2000a). Prabhananda and Hyde (1986) noted the potential utility of multifrequency SR measurements in distinguishing between possible mechanisms of spin lattice relaxation, although that capability was not available at that time. Spin lattice relaxation rates at 95 GHz (W-band),
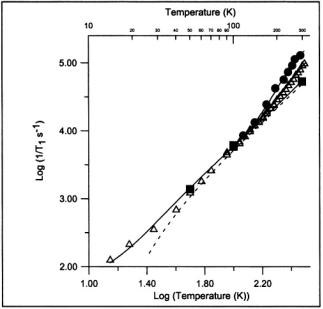
SATURATION RECOVERY EPR |
11 |
Figure 2. Temperature dependence of spin-lattice relaxation rates at W-band X-band
and S-band
in the perpendicular region of the spectrum for tempol doped 1:500 into 4- OH-2,2,6,6-tetramethyl-piperidine. The solid lines through the data are fit functions as described in Eaton et al. (2001), including a frequency-dependent contribution from an activated process that is attributed to methyl group rotation. The dashed line is the contribution to the fit function from the Raman process.
9.5 GHz (X-band), and S-band (3.2 GHz) as a function of temperature for 4- hydroxy-2,2,6,6-tetramethylpiperidinol-oxy (Tempol) doped into its diamagnetic analog are shown in Figure 2 (Eaton et al., 2001). The relaxation rate between 40 and 100 K is dominated by the Raman process and is independent of microwave frequency. Above about 130 K there is an additional contribution to the relaxation. If only data at X-band had been available, it would not have been possible to determine whether this additional contribution was due to a local vibrational mode or a thermally activated process. However, the multifrequency data show that the relaxation rate decreases in the order S-band > X-band > W-band. This frequency dependence is characteristic of a contribution from a thermallyactivated process, which can be described by a spectral density function, and inconsistent with a local mode (Eaton et al., 2001). The fit lines in Figure 2 were obtained with an activation energy of 1100 K (2.2 kcal/mole), which is
12 |
SANDRA S. EATON AND GARETH R. EATON |
in good agreement with that for rotation of nitroxyl methyl groups. for methyl rotation was obtained by ENDOR for a nitroxyl in a solid host (Barbon et al., 1999) and
to 2.3 kcal/mole (1030 to 1150 K) was obtained from the temperature dependence of spin echo dephasing for nitroxyls in glassy solutions (Tsvetkov and Dzuba, 1990; Shushkakov et al., 1989; Nakagawa et al., 1992). Thus, the multifrequency data support the proposal that rotation of the nitroxyl methyl groups at rates comparable to the electron Larmor frequency makes significant contributions to
at S- band and X-band in the motional regime that is present in the doped solid near room temperature. Work is currently underway to determine the importance of this contribution to
for nitroxyl radicals that are slowly tumbling in fluid solution.
5.2Oximetry – the Effect of Molecular Oxygen on
EPR oximetry is the measurement of oxygen concentration via the effect of oxygen on the EPR signal from a probe molecule. Many of these studies are based on linewidth changes or changes in CW power saturation curves. However, changes in are inherently more sensitive than CW lineshape to collisions with oxygen, and therefore SR is the preferred oximetry method when measurements of small changes are required (Hyde et al., 1990).
For nitroxyl spin labels in fluid solution, the time constant obtained in a long-pulse SR experiment is (Kusumi et al., 1982). In the absence of oxygen
was between about 1.5 and
for a range of spin labels and tumbling correlation times in membranes between 0 and 36 °C. The reciprocal of
for long-chain doxyl spin labels in lipid bilayers increased linearly with the partial pressure of oxygen when the gas surrounding a TPX capillary was changed from nitrogen to 100% oxygen at temperatures between 4 and 37° C. The oxygen transport parameter, W, was
defined as and was proposed as a monitor of the bimolecular collision rate between oxygen and the spin label (Kusumi et al., 1982). The oxygen transport parameter has been used to characterize the oxygen permeability of phosphatidylcholine-cholesterol membranes by varying the position of the spin label relative to the polar head groups (Subczynski et al., 1989). For the liquid-crystalline phase it was found that addition of cholesterol decreased oxygen transport in the membrane. In the absence of cholesterol, the incorporation of a double bond at the C9-C10 position of the alkyl chain in the lipid was found to decrease the oxygen transport parameter at all positions along the chain in phosphatidylcholine membranes (Subczynski et al., 1991). Values of W for a spin-labeled retinal analog incorporated into rhodopsin were 10 to 60 times smaller than in water and 1.1 to 20 times smaller than in model membranes, which indicates that
SATURATION RECOVERY EPR |
13 |
membrane proteins create significant resistance to the transport of molecular oxygen inside and across the membrane (Subczynski et al., 1992a). Studies of Chinese hamster ovary plasma membrane also indicated lower oxygen permeability for the membrane than for a comparable layer of water (Subczynski et al., 1992b). However, it was concluded that oxygen permeability of cell plasma membranes is not the rate-limiting step for cellular respiration.
In a series of measurements of oximetry, Hyde and coworkers explored the relative information content of three variants of the SR measurement, “short pulse,” “long pulse,” and “high observe power.” The recovery signal after a short saturating pulse is multi-exponential, and in favorable circumstances can distinguish several relaxation times: the electron the nitrogen nuclear
the spectral diffusion time, and/or the Heisenberg exchange rate (Yin et al., 1987). After a long saturating pulse, the various electron spin energy levels tend to be equalized, and the recovery tends to a single exponential, which is
Short and long are defined relative to the relevant relaxation times and motional regime. For nitroxyl radicals in fluid solution, relaxation times are fast enough that a
pulse (Kusumi et al., 1982) or
pulse (Fajer et al., 1986) is “long.” Similarly, high observe power is the power at which the second term in the expression
cannot be ignored, i.e. the saturation factor (eq. (1)) is significantly less than 1. Usually, one performs SR measurements with very low observing power to avoid artificial shortening of the measured
Lower observing power usually results in poorer signal-to-noise. However, Hyde et al. (1990) showed that if the goal is to measure the oxygen transport parameter, high observing powers can be used to measure an effective
at two oxygen concentrations.
In a short-pulse SR experiment the recovery time constant for nitroxyl spin labels in fluid solution has contributions from (i) Heisenberg exchange due to collisions between spin labels and collisions between oxygen and spin labels, (ii) nitrogen nuclear spin relaxation and (iii) the intrinsic for the spin label in the absence of oxygen (Yin and Hyde, 1987a). The effects of the multiple contributions to the recovery curves can be interpreted in terms of a model based on population differences between electron spin energy levels. Experimental SR curves can be analyzed in terms of multiple exponentials (Yin and Hyde, 1987a,b). In 1-palmitoyl-2-oleoylphospha- tidylcholine bilayers containing a transmembrane
peptide the oxygen transport parameter was substantially higher in the middle of the bilayer than on the edges (Subczynski et al., 1998). Analysis of short-pulse SR data has been used to identify regions of a bacteriorhodopsin-rich reconstituted membrane in which oxygen transport is a factor of 5 slower than in the bulk (Ashikawa et al., 1994). It was speculated that these domains consist of lipids in contact with two proteins and/or in contact with
14 |
SANDRA S. EATON AND GARETH R. EATON |
protein and boundary lipids. In influenza viral membrane the oxygen transport rate in the slow-oxygen-transport domains is a factor of two smaller than in purple membrane, where bacteriorhodopsin is aggregated, which suggests that the slow oxygen transport regime in the viral membrane is a cholesterol-rich raft domain (Kawasaki et al., 2001). The contributions to the recovery curve from nitroxyl-oxygen collisions were distinguished from nitroxyl-nitroxyl interactions by varying oxygen and nitroxyl concentrations.
5.3Heisenberg Exchange due to Nitroxyl-nitroxyl Collisions
The decrease in (increase in
due to nitroxyl-nitroxyl collisions has been used to characterize lipid dynamics using stearic acids spin-labeled at various positions along the chain. The nitroxyl Heisenberg exchange rates determined by analysis of short-pulse SR were shown to be proportional to spin label concentration, and were judged to be valid indicators of bimolecular collision rates (Yin et al., 1987). The use of
and
labels combined with short-pulse SR overcomes spectral overlap problems that are encountered with CW methods of measuring Heisenberg exchange (Yin et al., 1988). SR studies of
and
labeled stearic acid pairs in model membranes prepared from lipids with varying chain lengths showed little dependence of lateral diffusion on chain length (Feix et al., 1987). For doxylstearates labeled at the 5-, 12-, or 16positions, collision frequencies were higher in dimyristoyl-phosphatidylcholine (DMPC) relative to that in dielaidoylphosphatidylcholine (DEPC), consistent with CW studies that showed increased order in DEPC than in DMPC (Yin et al., 1990). Addition of cytochrome c to cardiolipin bilayers caused an increase in
that was attributed to changes in the molecular dynamics in the vicinity of double bonds in the acyl chains of the bilayer (Pinheiro et al., 1993). The effects of lutein and cholesterol on collision frequencies among doxyl-stearic acids in lipid bilayers confirmed the occurrence of vertical fluctuations of alky chain ends near the bilayer surface (Yin and Subczynski, 1996).
5.4Distance Between a Radical and a More Rapidly Relaxing Metal Ion
The enhancement in the spin lattice relaxation rate for a slowly relaxing spin due to dipolar interaction with a more rapidly relaxing metal ion can be analyzed to determine the distance between the two paramagnetic centers. This major application of SR was recently reviewed by Eaton and Eaton (2000b) and applications in photosynthesis were reviewed by Lakshmi and
SATURATION RECOVERY EPR |
15 |
Brudvig (2000). An example of the use of SR to determine the distance between the Fe(III) in metmyoglobin and a spin label is discussed in ch. 8 of this volume.
6.PROGNOSIS
Until recently SR experiments could be performed only on locallyconstructed spectrometers. The recent addition of an option for SR to the Bruker Elexsys bridge will make the technique more widely available. We expect that SR will be much more widely used in the future due to the availability of commercial instrumentation.
7.ACKNOWLEDGMENT
Our work in this area is supported by National Institutes of Health Grant EB002807 (formerly GM21156).
8.REFERENCES
Ashikawa, I., Yin, J.-J., Subczynski, W. K., Kouyama, T., Hyde, J. S., and Kusumi, A. (1994). Molecular organization and dynamics in bacteriorhodopsin-rich reconstituted membranes: discrimination of lipid environments by the oxygen transport parameter using pulse ESR spin-labeling technique. Biochemistry 33, 4947-4952.
Barbon, A., Brustolon, M., Maniero, A. L., Romanelli, M., Brunel, L.-C. (1999). Dynamics and spin relaxation of tempone in a host crystal. An ENDOR, high-field EPR, and electron spin echo study. Phys. Chem. Chem. Phys. 1, 4015-4023.
Beck, W. F., Innes, J. B., Lynch, J. B., and Brudvig, G. W. (1991). Electron spin-lattice relaxation and spectral diffusion measurements on tyrosine radicals in proteins. J. Magn. Reson. 91, 12-29.
Bloembergen, N. (1949) On the interaction of nuclear spin in a crystalline lattice. Physica 15, 386-426.
Bloembergen, N. and Wang, S. (1954) Relaxation effects in paraand ferromagnetic resonance. Phys. Rev. 93, 72-83.
Bowers, K. D., and Mims, W. B. (1959) Paramagnetic relaxation in nickel fiuosilicate. Phys. Rev. 115, 285-295.
Collins, S. A., Kyhl, R. L., and Strandberg, M. P. W. (1959) Spin lattice relaxation from state of negative susceptibility. Phys. Rev. Lett. 2, 88-90.
Daraseliya, D. M. and Manenkov, A. A. (1970) Quenching of cross correlation in inhomogeneously broadened epr lines. JETP Letters 11, 224-226 (337-339 in Russian)
Daraseliya, D. M., Epifanov, A. S., and Manenkov, A. A. (1970) Relaxation in inhomogeneously broadened EPR lines. Soviet Physics JETP 32, 244-249 (59, 445-456 in Russian).

16 SANDRA S. EATON AND GARETH R. EATON
Davis, C. F. Jr., Strandberg, M. W. P., and Kyhl, R. L. (1958) Direct measurement of electron spin-lattice relaxation times. Phys. Rev. 111, 1268-1272.
Eaton, S. S. and Eaton, G. R. (2000a). Relaxation times of organic radicals and transition
metal ions, Biol. Magn. Reson. 19, 29-154. |
|
|
Eaton, S. S., and Eaton, G. R. (2000b). Determination of distances based on |
and |
Biol. |
Magn. Reson. 19, 347-381
Eaton, S. S., Harbridge, J., Rinard, G. A., Eaton, G. R., and Weber, R. T. (2001) Frequency dependence of electron spin relaxation for three S=1/2 species doped into diamagnetic solid hosts, Appl. Magn. Reson. 20, 151-157.
Fajer, P., Thomas, D. D., Feix, J. B., and Hyde, J. S. (1986). Measurement of rotational molecular motion by time-resolved saturation transfer electron paramagnetic resonance,
Biophys.J. 50, 1195-1202.
Feix, J. B., Yin, J. J., and Hyde, J. S. (1987) Interactions of nitrogen-14:nitrogen-15 stearic acid spin-label pairs: effects of host lipid alkyl chain length and unsaturation.
Fessenden, R. W., Hornak, J. P., and Venkataraman, B. (1981) Electron spin-lattice relaxation of transient free radicals. J. Phys. Chem. 74, 3694-3704.
Freed, J. H. (1974) Theory of saturation and double resonance in electron spin resonance spectra. VI. Saturation recovery. J. Phys. Chem. 78, 1156-1167.
Giordmaine, J. A., Alsop, L. E., Nash, F. R., and Townes, C. H. (1958) Paramagnetic relaxation at very low temperature. Phys. Rev. 109, 302-311.
Harbridge, J. R., Eaton, S. S., and Eaton, G. R. (2002) Electron spin lattice relaxation in radicals containing two methyl groups, generated by of polycrystalline solids,
J. Magn. Reson. 159, 195-206.
Hausser, K. H. and Brunner, H. (1998) The Effect of concentration and oxygen in EPR, in Foundations of Modern EPR, G. R. Eaton, S. S. Eaton, and K. Salikhov, eds., World Scientific, Singapore, 469-481.
Huisjen, M. and Hyde, J. S. (1974a) Saturation recovery measurements of electron spin-lattice relaxation times of free radicals in solution. J. Chem. Phys. 60, 1682-1683.
Huisjen, M. and Hyde, J. S. (1974b) A pulsed EPR spectrometer. Rev. Sci. Instrum. 45, 669675.
Hyde, J. S. (1979) Saturation recovery methodology, in Kevan, L., and Schwartz, R. N., eds.,
Time Domain Electron Spin Resonance, Wiley-Interscience, New York.
Hyde, J. S., Yin, J.-J., Feix, J. B., and Hubbell, W. L. (1990) Advances in spin label oximetry.
Pure & Appl. Chem. 62, 255-260.
Hyde, J. S. (1998) Saturation recovery in Foundations of Modern EPR, G. R. Eaton, S. S. Eaton, and K. Salikhov, eds., World Scientific, Singapore, 607-618.
Kawasaki, K., Yin, J.-J., Subczynski, W. K., Hyde, J. S., and Kusumi, A. (2001). Pulse EPR detection of lipid exchange between protein-rich raft and bulk domain in the membrane: methodology develoment and it application to studies of influenza viral membrane.
Biophys. J. 80, 738-748.
Kusumi, A., Subczynski, W. K., and Hyde, J. S. (1982) Oxygen transport parameter in membranes deduced by saturation recovery measurements of spin-lattice relaxation times of spin labels. Proc. Natl. Acad. Sci. USA 79, 1854-1858.
Lakshmi, K. V., and Brudvig, G. W. (2000) Electron paramagnetic resonance distance measurements in photosynthetic reaction centers, Biol. Magn. Reson. 19, 513-567.
Lingam, K. V., Nair, P. G., and Venkataraman, B. (1972) Spin lattice relaxation studies of semiquinone ions, Proc. Indian Acad. Sci. A 76, 207220.
Mailer, C., Danielson, J. D. S., and Robinson, B. H. (1985) Computer-controlled pulsed electron-paramagnetic-resonance spectrometer. Rev. Sci. Instrum. 56, 1917-1925.
SATURATION RECOVERY EPR |
17 |
Manenkov, A. A. and Milyaev, V. A. |
(1970) Paramagnetic relaxation processes in |
single crystals at helium temperatures. Soviet Physics JETP 31, 427428 (58, 796-799 in Russian)
Manenkov, A. A. and Pol’skii, Yu. E. (1964) Relaxation processes in the paramagnetic resonance of in
Soviet Physics JETP 45, 1425-1429 (1963) (18, 985-987 (1964)in transl.)
Manenkov, A. A. and Prokhorov, A. M. (1962) SpinlLattice relaxation and cross-relaxation interactions in chromium corundum. Soviet Physics JETP 42, 75-83 (54-59 in translation).
Manenkov, A. A., Milyaev, V. A. and Prokhorov, A. M. (1962) The relaxation time of and
ions in single crystals of rutile. Soviet Physics Solid State 4, 388-391 (280-283 in translation).
Nakagawa, K., Candelaria, M. B., Chik, W. W. C., Eaton, S. S., and Eaton, G. R. (1992). Electron spin relaxation times of chromium(V). J. Magn. Reson. 98, 81-91.
Pace, J. H., Sampson, D. F., and Thorp, J. S. (1960). Phys. Rev. Lett. 4, 18-19.
Pastor, R. C. and Hoskins, R. H. (1960) Paramagnetic resonance in charred dextrose. J. Chem. Phys. 32, 264-269.
Percival, P. W. and Hyde, J. S. (1975) Pulsed EPR spectrometer II. Rev. Sci. Instrum. 46, 1522-1529.
Percival, P. W. and Hyde, J. S. (1976) Saturation-recovery measurements of the spin-lattice relaxation times of some nitroxides in solution. J. Magn. Reson. 23, 249-257.
Pinheiro, T. J. T., Bratt, P. J., Davis, I. H., Doetschman, D. C., and Watts, A. (1993). Spinlattice relaxation times of phospholipid aminoxyl spin labels in cardiolipin-cytochrome c bilayers: a pulse saturation recovery study, J. C. S. Perkin Trans 2, 2113-2117.
Prabhananda, B. S., and Hyde, J. S. (1986). Study of molecular motion in liquids by electron spin relaxation: halogenated p-semiquinone anions in alcohols, J. Chem. Phys. 85, 67056712.
Quine, R. W., Eaton, S. S. and Eaton, G. R. (1992) A saturation recovery electron paramagnetic resonance spectrometer. Rev. Sci. Instrum. 63, 4251-4262.
Quine, R. W., Rinard, G. A., Ghim, B. T., Eaton, S. S., and Eaton, G. R. (1996) A 1-2 GHz pulsed and continuous wave electron paramagnetic resonance spectrometer. Rev. Sci. Instrum. 67, 2514-2527.
Rengen, S. K., Khakhar, M. P., Prabhananda, B. S., and Venkataraman, B. (1972) Electron spin-lattice relaxation in organic free radicals in solution. Pure & Appl. Chem. 32, 287305.
Rengen, S. K., Khakhar, M. P., Prabhananda, B. S., and Venkataraman, B. (1974a) Study of molecular motions in liquids by electron spin lattice relaxation measurements. I. Semiquinone ions in hydrogen bonding solvents. Pramana 3, 95-121.
Rengen, S. K., Khakhar, M. P., Prabhananda, B, S., and Venkataraman, B. (1974b) Study of molecular motions in liquids by electron spin lattice relaxation measurements. II. 2,5-Di- tert-butylsemiquinone Ion in Acetonitrile and Tetrahydrofuran. J. Magn. Reson. 16, 35-43.
Rengen, S. K., Bhagat, V. R. Sastry, V. S. S., and Venkataraman, B. (1979). Magnetic fieldpulsed ELDOR spectroscopy, J. Magn. Reson. 33, 227-240.
Scott, P. L., and Jeffries, C. D. (1962). Spin-lattice relaxation in some rare-earth salts at helium temperature; observation of the phonon bottleneck, Phys. Rev. 127, 32-51.
Shushkakov, O. A., and Dzuba, S. A. (1989). Barriers for internal rotation of methyl groups screening nitroxide paramagnetic fragments as determined by an electron spin-echo technique, J. Struct. Chem. 30, 75-80 (1989).
Standley, K. J., and Vaughan, R. A. (1969) Electron Spin Relaxation Phenomena in Solids,
Plenum Press, N. Y.

18 |
SANDRA S. EATON AND GARETH R. EATON |
Subczynski, W. K., Hyde, J. |
S., and Kusumi, A. (1989). Oxygen permeability of |
phosphtidylcholine-cholesteral membranes, Proc. Natl. Acad. Sci. (U.S.) 86, 4474-4478. Subczynski, W. K., Hyde, J. S., and Kusumi, A. (1991). Effect of alkyl chain unsaturation and
cholesterol intercalation on oxygen transport in membranes: a pulsed ESR spin labelling study, Biochemistry 20, 8578-8590.
Subczynski, W. K., Renk, G. E., Crouch, R. K., Hyde, J. S., and Kusumi, A. (1992a). Oxygen diffusion-concentration product in rhodopsin as observed by a pulse ESR spin labelling method, Biophys. J. 63, 573-577.
Subczynski, W. K., Hopwood, L. E., and Hyde, J. S. (1992b) Is the mammalian cell plasma membrane a barrier to oxygen transport? J. Gen. Physiol. 100, 69-87.
Subczynski, W. K., Lewis, R. N. A. H., McElhaney, R. N., Hodges, R. S., Hyde, J. S., and
Kusumi, A. (1998) Molecular organization and dynamics of a |
1-palmitoyl-2- |
oleoylphosphatidylcholine bilayer containing a transmembrane |
peptide, |
Biochemistry 37, 3156-3164. |
|
Tsvetkov, Yu. D., and Dzuba, S. A. (1990). Pulsed EPR and molecular motion, Appl. Magn. Reson. 1, 179-194.
Venkataraman, B. (1982) Time resolved electron spin resonance spectroscopy. Current Science 51, 397-400.
Weissman, S. I., Feher, G., and Gere, E. A. (1957) The spin relaxation time of triphenylmethyl at low temperatures. J. Am. Chem. Soc. 79, 5584-5585.
Yin, J.-J., and Hyde, J. S. (1987a) Spin label saturation-recovery electron spin resonance measurements of oxygen transport in membranes. Z. Physikal. Chem. Neue Folge 153, 5765.
Yin, J.-J., and Hyde, J. S. (1987b) Application of rate equations to ELDOR and saturation recovery experiments on spin-label pairs, J. Magn. Reson. 74, 82-93.
Yin, J.-J., and Hyde, J. S. (1989) Use of high observing power in electron spin resonance saturation-recovery experiments in spin-labeled membranes. J. Chem. Phys. 91, 60296035.
Yin, J.-J., Feix, J. B., and Hyde, J. S. (1988) Solution of the nitroxide spin-label spectral overlap problem using pulse electron spin resonance. Biophys. J. 53, 525-531.
Yin, J.-J., Feix, J. B., and Hyde, J. S. (1990) Mapping of collision frequencies for stearic acid spin-labels by saturation-recovery electron paramagnetic resonance. Biophys. J. 58, 713720.
Yin, J.-J., Pasenkiewicz-Gierula, M., and Hyde, J. S. (1987) Lateral diffusion of lipids in membranes by pulse saturation recovery electron spin resonance. Proc. Natl. Acad. Sci. USA 84, 964-968.
Yin, J.-J., and Subczynski, W. K. (1996). Effects of lutein and cholesterol on alkyl chain bending in lipid bilayers: a pulse electron spin resonance spin labeling study. Biophys. J. 71, 832-839.