
Genomics: The Science and Technology Behind the Human Genome Project. |
Charles R. Cantor, Cassandra L. Smith |
|
Copyright © 1999 John Wiley & Sons, Inc. |
|
ISBNs: 0-471-59908-5 (Hardback); 0-471-22056-6 (Electronic) |
6 Genetic Analysis
WHY WE NEED GENETICS
Despite the great power of molecular biology to examine the information coded for by DNA, we have to know where on the DNA to look to find information of relevance to particular phenomena. Some genes have very complex phenotypic consequences. We may
never have the ability to look at a DNA sequence and infer, directly, that it regulates some aspect of facial features or mathematical reasoning ability. Genetic analysis offers a totally independent approach to determining the location of genes responsible for inherited traits. In this chapter we will explore the power of this approach and also some of its particular limitations. Genetics, in the human, will mostly serve as a low resolution method for localizing genes, but it is the only method available if the only prior information about a gene is some hypothesis about its function, or some presumed phenotype that results from a particular DNA sequence (allele) of that gene.
BASIC STRATEGY FOR GENETIC ANALYSIS IN THE HUMAN:
LINKAGE MAPPING
In most organisms, genetics is carried out by breeding specific pairs of parents and exam- |
|
|||||
ining the characteristics of their offspring. Clearly this approach is not practical in the hu- |
|
|||||
man. Even leaving aside the tremendous ethical issues such an approach would raise, the |
|
|
||||
small size of our families, and the long lifespan of our species, make genetic experimen- |
|
|||||
tation all but impossible. Instead, what must be done is to perform retrospective analyses |
|
|||||
of inheritance in families. Statistical analysis of |
the pattern of inheritance is used |
|
instead |
|
||
of direct genetic manipulation to test hypotheses about the genetic mechanism underlying |
|
|
|
|||
a particular trait. |
|
|
|
|
|
|
Human beings and other mammals are diploid organisms. They contain two copies of |
|
|
||||
each homologous chromosome. Normally an offspring receives one of its homologs from |
|
|
|
|||
each parent (Fig. 6.1). This is accomplished by a specialized cell division process, meio- |
|
|||||
sis, which occurs during the formation of sperm and eggs, a process known as gametoge- |
|
|
|
|||
nesis. Sperm cells and egg cells (ova) are haploid; both contain only a single one of each |
|
|||||
of the homologous pairs of the parental chromosomes. When sperm and egg combine in |
|
|
|
|||
fertilization, this reconstitutes a diploid cell |
which is |
a mosaic of halves |
of the |
genomes |
|
|
of its parents. |
|
|
|
|
|
|
Linkage is the tendency for two observable genetic traits, called |
|
|
markers, |
to be coin- |
||
herited if they lie near each other on the same chromosome. To be distinguishable genet- |
|
|||||
ically, a marker must occur in more than one form (e.g., eye color) in different members |
|
|||||
of the population. We call these forms alleles. |
Consider the case shown in Figure |
6.1 |
|
|||
where two detectable markers, one with alleles A or a and the other with alleles B |
or b, |
|
||||
are being followed in a family. Markers A and |
B are |
both inherited from |
the |
father. |
|
165

166 GENETIC ANALYSIS
Figure 6.1 Basic scheme for chromosome segregation in the genetics of diploid organisms. |
(a) |
||
parental genome. |
(b) Possible gametes. |
(c) Possible offspring (all equally |
probable). |
Markers on different chromosomes would, |
by chance, pass |
together |
to an |
offspring |
|
||||
only 25% of the time, since there is a 50% chance of the offspring receiving each partic- |
|
||||||||
ular homologous chromosome. In contrast, |
markers on the same chromosome will al- |
|
|||||||
ways be coinherited, so long as the chromosome remains intact. Half of the offspring in |
|
||||||||
this case would receive both allele A and allele B. This simple picture of inheritance is |
|
||||||||
complicated by the process known as |
|
|
|
|
meiotic recombination. |
During the formation of |
|||
sperm and eggs, at one stage in meiosis, |
homologous chromosomes pair up physically, |
|
|||||||
and the DNA molecules within these |
chromosomes |
can |
form |
a four-ended structure |
|
||||
known as a |
Holliday junction. |
|
This structure can be reverted to unpaired DNA duplexes |
||||||
in two different ways. Half the time the result is to produce a DNA molecule that con- |
|
||||||||
sists of part of each parental DNA, as shown in Figure 6.2. When these rearranged chro- |
|
||||||||
mosomes are passed to offspring, the result is that part of each parental homolog is in- |
|
||||||||
herited. This can separate two markers that were originally linked on one of the parental |
|
||||||||
chromosomes. |
|
|
|
|
|
|
|
|
|
Meiotic recombination in the human |
occurs at least once for each pair of homolo- |
|
|||||||
gous chromosomes. |
1 If |
two |
markers |
A |
and B |
are 1 Mb |
apart, |
there is roughly |
a 1% |
1 It is interesting to speculate why meiotic recombination appears to be obligatory. In the absence of recombination, a chromosome that acquired a dominant lethal mutation would be lost to all future generations. Alleles that accidentally resided on this chromosome would also be lost. The resulting drift toward homozygosity could be harmful from an evolutionary standpoint. This drift is largely prevented by the requirement for meiotic recombination.
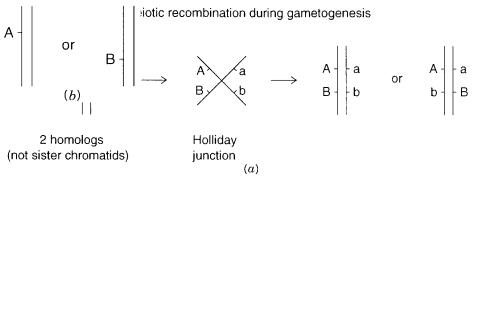
BASIC STRATEGY FOR GENETIC ANALYSIS IN THE HUMAN: LINKAGE MAPPING |
167 |
Figure 6.2 |
Minimalistic view of meiotic recombination in a single |
|
pair of homologous chromosomes. |
(a) Formation and resolution of a |
|
Holliday junction. |
(b) |
Typical offspring after a recombination event. |
chance that they will be separated, and an offspring will be produced with A and b |
not |
|
|
|
|||||||||
B, and vice versa. Because human families are generally small, it becomes very diffi- |
|
|
|
||||||||||
cult to |
detect recombination events for closely linked markers. In |
other |
words, |
the res- |
|
|
|||||||
olution of genetics in the human is quite limited. It would take families with 100 off- |
|
|
|||||||||||
spring to detect a single recombination event that would place two genes 1 Mb apart. |
|
|
|
||||||||||
Since such families are unavailable, one must pool data from differ |
|
|
|
|
ent fam |
ilies in order |
|
||||||
to have a large enough population to provide a reasonable chance of seeing a rare re- |
|
|
|
||||||||||
combination event. Such pooling makes the presumption that markers A and B in dif- |
|
|
|
||||||||||
ferent fam |
ilies actually correspond to the same |
genes. For many reasons this |
is not |
al- |
|
|
|||||||
ways the case. For example, if the marker is the elimination of a complex metabolic |
|
|
|
||||||||||
pathway, it is possible |
that the phenotype might be |
similar |
regardless |
of |
which |
gene |
|
|
|
||||
for one of the enzymes in the pathway was inactivated. Such multigenic complications |
|
|
|
||||||||||
may be quite common in |
human genetics, and they can |
severely |
impair |
our |
ability |
to |
|
|
|
||||
use linkage analysis to find genes. |
|
|
|
|
|
|
|
|
|
||||
|
An additional complication is the restricted and uncontrolled variability of |
human |
|
|
|
||||||||
DNA. In order for a marker to be useful, one must be |
able to distinguish which of the |
|
|
|
|||||||||
two parental homologous chromosomes has been inherited. If both chromosomes of a |
|
|
|
|
|||||||||
parent contain the same marker A, there is no way to tell from this marker alone which |
|
|
|
||||||||||
chromosome |
the offspring received. We say that |
this |
marker |
is |
uninformative. |
|
|
|
|||||
If |
both |
parents |
have |
the same set of allele pairs, A and a, as |
shown |
in |
|
|
Figure |
6.3, |
|||
the child can have allele pairs A, A or a, a or A, a. Once again we have no way of dis- |
|
|
|
||||||||||
cerning from this marker alone which chromosome came from which parent. To carry |
|
|
|
|
|||||||||
out |
human |
genetics |
ef |
fectively, it is |
necessary to have large numbers of |
markers which |
|
|
|||||
are informative. That is, at any particular region, |
we would like to be able to distin- |
|
|
||||||||||
guish easily among the four homologous chromosomes originally carried by the two |
|
|
|
||||||||||
parents. |
|
|
|
|
|
|
|
|
|
|
|
|
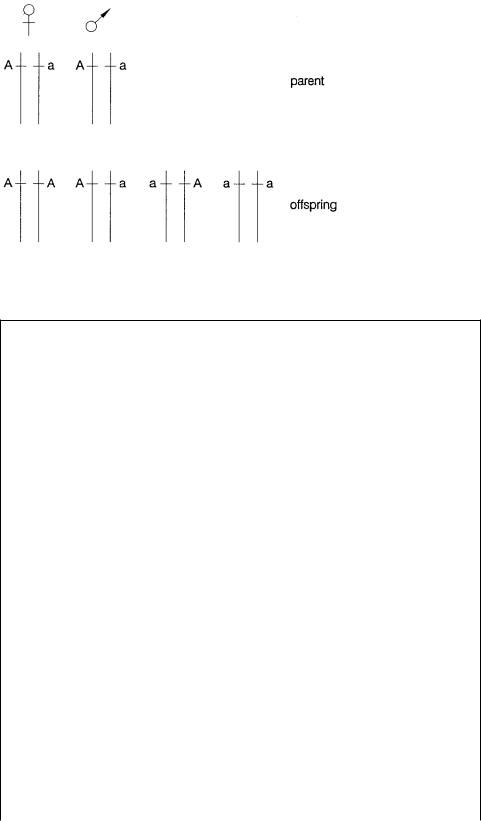
Figure 6.3 Examples of cases where particular combinations of alleles in parents and offspring confound attempts to trace genetic events uniquely.
BOX 6.1
MEIOSIS
The process of meiosis and the recombination events that occur during meiosis, are actually much more complicated than the simple picture given in the text. Figure 6.4 illustrates this process for the relatively simple case of a cell with only two different chromosomes. The parental cell is diploid; it contains two copies of each homologous chromosome. When this cell duplicates its genome, in preparation for cell division, it
becomes |
tetraploid. At this point each chromosome |
exists as a pair of sister chro- |
matids. |
During the first meiotic cell division, the |
homologous chromosomes line up |
and associate, as shown in Figure 6.4. The resulting structures are pairs of homologous chromosomes, each containing two paired sister chromatids (identical copies of each other). Thus, altogether, four DNA molecules (eight DNA strands!) are involved in close proximity. In order for these molecules to separate, there appears to be an obligatory recombination event that involves at least one chromatid from each pair of the homologous chromosomes. As shown in Figure 6.4, this results in a reciprocal exchange
of DNA between two chromatids, while, in the simple case illustrated, the other two chromatids remain unaltered.
The homologous chromosomes segregate to separate daughter cells during the last stages of the first meiotic division. When this is completed, the result is two diploid cells as in ordinary mitotic division. However, what is profoundly different about these cells is that each contains paired homologous chromosomes that now consist of some regions where both copies of the homolog arose by duplication of a single one of the initial homologous pairs. Because of recombination, one member of each chromosome
pair at this point contains some material that is a mosaic of the two original parental homologs. In the second meiotic cell division, each the pair of homologous chromosomes is segregated into a separate daughter cell that goes on, eventually, to become a
haploid gamete. Thus, as |
shown in Figure 6.4, some gametes |
receive chromosomes |
||
that |
are unaltered versions of the original parental material. |
Others receive |
chromo- |
|
somes that have been rearranged by meiotic recombination. The |
true picture is |
even |
||
more |
complex than this, and |
we will return to it later. |
|
|

BOX 6.1 |
(Continued) |
Figure 6.4 Some of the steps in meiosis, illustrated for a hypothetical cell with only two types of chromosomes.
169

170 GENETIC ANALYSIS
A GLOSSARY OF GENETIC TERMS
The science of genetics emerged and matured before DNA was known to be the genetic material. Many terms and concepts were coined without a knowledge of their chemical basis. Here we define some of the commonly used genetic terms needed to follow arguments in human genetics, and we provide an indication of their molecular basis. The first few of these notions are illustrated schematically in Figure 6.5.
Gene. A commonly used term for a unit of genetic information sufficient to determine an observable trait. In more contemporary terms, most genes determine the sequence and structure of a particular protein molecule. However, genes may code for untranslated RNAs like tRNA or rRNA, or they may code for more than one different protein when complex mRNA processing occurs.
Locus. A site on a chromosome, that is, site on a DNA molecule.
Allele. A variant at a particular locus, namely a DNA sequence variant (simple or complex) at the particular locus of interest.
Homozygosity. The two homologous chromosomes in a diploid cell have the same allele at the locus of interest.
Heterozygosity. |
The two homologous chromosomes in a diploid cell have different al- |
|||
leles at the locus of interest. |
|
|
||
Independent |
segregation. |
The notion that each of |
the parental homologous chromo- |
|
somes in |
a diploid |
cell has an |
equal chance of being passed to |
a particular daughter |
cell or offspring. Thus, considering only two chromosomes in Figure 6.6, there will be four possible types of gametes (neglecting recombination), and each of these will occur at equal frequencies.
Figure 6.5 Some simple |
ideas in |
classical genetics. |
(a) Locus. |
(b) |
|
Allele. |
(c) Homozygote. |
(d) |
Heterozygote. |
|
|
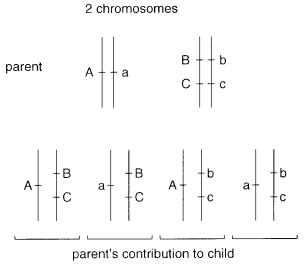
A GLOSSARY OF GENETIC TERMS |
171 |
Figure 6.6 Independent segregation of alleles on two different chromosomes. Shown are parental genomes and possible gametes arising from these genomes.
Phenotype. What one sees, namely the complete set of observable inherited characteristics of an organism, or the particular set of characteristics controlled by the locus or loci of interest.
Genotype. What one gets, namely the genetic constitution, which is the particular set of alleles inherited by the organism as a whole, or for the particular locus or loci of interest.
Haplotype. The actual pattern of alleles on one chromosome, that is, on a single DNA molecule.
Dominant. An allele that produces an identical phenotype if it is present on one homologous chromosome, or on both. True simple dominant traits are rare in the human; one example may be Huntington’s disease where individuals carrying one or two disease alleles appear to have indistinguishable disease phenotypes.
Recessive. |
An allele that produces a particular phenotype only if it is present on both ho- |
|
mologous |
chromosomes. Obviously dominant and recessive are oversimplifications |
|
that ignore molecular mechanisms and the possibility of more than two alleles at a lo- |
||
cus. In principle, with three alleles, A, |
a, and a,˙ there are six possible genotypes: AA, |
|
Aa, A˙ |
a, aa, a˙ a, and ˙ a˙a, and each |
of these might yield a distinguishable phenotype if we |
use precise enough definitions and measurements.
Penetrance. The chance that an allele yields the expected phenotype. This term covers a multitude of sins and oversimplifications. In simple genetic systems the penetrance should normally be 1.0. In outbred species like the human, there can be unknown ge-
netic variants that interact with the gene product at the locus in question and produce different phenotypes in different individuals. Purely at the DNA level we expect all phenotypes to be distinguishable and to show complete penetrance because the pheno-
type at the level of DNA is identical to the genotype. An exception occurs if the phenotype requires the expression of a particular allele in an environmental context that
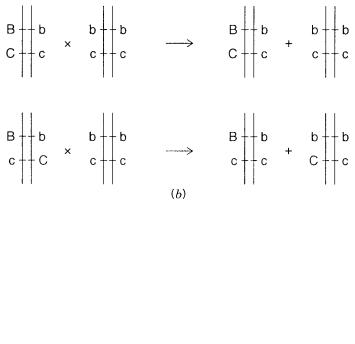
172 GENETIC ANALYSIS
might not be experienced by all individuals. For example, an allele that determined a serious adult emotional illness might require certain physical or mental stress during the development of the nervous system in order to actually result in phenotypically detectable disease.
Phase. |
The distribution of particular alleles on homologous chromosomes. Suppose that |
||
alleles B and b can occur at |
one locus, alleles C and c at another. An individual with |
||
the phenotype BbCc can have two possible genotypes, as shown in Figure 6.7 |
a . These |
||
are arbitrarily called cis and trans in analogy with chemical isomers. The two possible |
|||
phases in this simple case can |
be distinguished easily by performing a genetic |
cross. |
|
An individual with phenotype BbCc is mated with an individual like bbcc (or BBCC). |
|||
As shown in Figure 6.7 |
b , this allows an unambiguous phase determination. In humans, |
where we cannot control mating, we need to find family members whose particular inheritance pattern allows an unambiguous determination of the phase of markers to be determined. This is not always possible, and one must frequently make unprovable assumptions about recombination (or the lack of it) in the family in order to assess the phase.
Linkage. A statement that alleles at two loci, say B and C, tend to be coinherited. If linkage is complete they will be coinherited 100% of the time. In molecular terms, these alleles occur on loci that lie near to each other on the same DNA molecule.
Linkage group. A set of loci that in general tend to be inherited together. In effect, a linkage group is a chromosome, a set of genes on a single DNA molecule.
Figure 6.7 |
Phase of alleles at two loci on the same chromosome. |
(a) Definition of two possible |
|
phases, arbitrarily |
called cis and trans. |
(b) Determination of |
the phase in a particular individual by |
performing a genetic cross with an appropriately informative partner. |
|
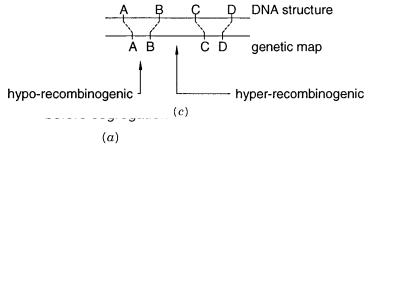
A GLOSSARY OF GENETIC TERMS |
173 |
Unlinked loci. Two loci that show random cosegregation. Ordinarily, if an allele at one locus, A, is inherited, there is a 50% chance that an allele, B, at another unlinked locus will also be inherited. Loci on different (nonhomologous) chromosomes behave as un-
linked. So do loci very far apart on the same large chromosome.
Meiotic recombination. |
A process, already described at the chromosomal level in Box |
||
6.1, that makes the description of genetic inheritance much more complex. However, it |
|||
is also the major event that allows us to map the location of genes. Simply put, meiotic |
|||
recombination is the breakage and reunion of DNA molecules prior |
to their segrega- |
||
tion to daughter cells during the process of gamete formation. Because of meiotic re- |
|||
combination, alleles at linked loci may occasionally fail to show co-segregation (Fig. |
|||
6.8 a ). In the human genome, meiotic recombination occurs at a frequency of about 1% |
|||
for two |
loci spaced 1 |
Mb apart. The actual frequency observed |
between two loci is |
called |
. We define a 1% recombination frequency as 1 cM (centiMorgan after the ge- |
||
neticist |
Morgan). Most human chromosomes are more than 100 |
cM in length; thus |
|
genes at opposite ends of these chromosomes behave as unlinked. Note that recombi- |
|||
nation is an obligatory event for each pair of homologs in meiosis. This implies that |
|||
smaller chromosomes may have overall higher average levels of recombination. |
|||
Genetic map. |
An ordered set of loci with relative spacing (and order) determined from |
||
measured |
recombination frequencies. The simplest map construction |
would be based |
|
on pairwise recombination frequencies (Fig. 6.8 |
b ). However, more accurate maps can |
||
be made |
by considering |
the inheritance of multiple loci simultaneously. This allows |
|
for multiple crossovers in recombination, multiple DNA breaks and joins, to be con- |
|||
sidered. Frequently genetic maps are displayed as statements about the relative likeli- |
|||
hood of the order shown. For each pair of adjacent loci, what is presented is the rela- |
|||
tive odds in favor of |
the order shown, say ABCD, as opposed to an inverted order, |
Figure 6.8 |
Genetic |
maps constructed from recombination frequencies between pairs of adjacent |
|
|||
loci. |
(a) |
Effect |
of recombination on |
two adjacent loci before segregation. |
(b) Genetic map con- |
|
structed |
from |
recombination |
frequencies. |
(c) Typical relationship between a genetic map |
and the |
underlying structure of the DNA, a physical map.
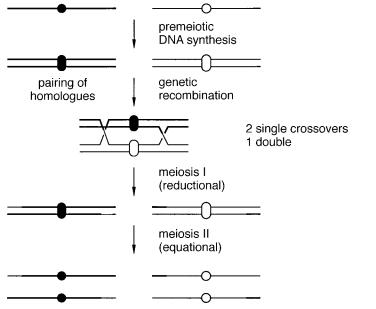
174 |
GENETIC |
ANALYSIS |
|
|
|
||
|
ACBD, given the available recombination data. In practice, the current human genetic |
||||||
|
map has an average spacing between markers of around 2 |
cM. The ultimate map we |
|||||
|
are likely to achieve in the absence of significant improvements in our analytical meth- |
||||||
|
ods is about a 1 cM map (see Fig. 6.29 |
|
c ). |
||||
RELATIONSHIP |
BETWEEN |
THE |
PHYSICAL |
AND THE GENETIC |
MAPS |
|
|
The |
order of |
loci must be |
the same |
on the genetic map |
and on |
its corresponding physical |
map. Both must ultimately reflect the DNA sequence of the chromosome. However, the genetic map is based on recombination frequencies, while the physical map is based directly on measurements of DNA structure. The meiotic recombination frequency is not uniform along most chromosomes. Regions are observed where recombination is much more frequent than average, hot spots, and where recombination is much less frequent than average, cold spots.
Such |
regions |
lead |
to considerable |
metrical distortion |
when genetic and physical maps are |
laid |
side by |
side |
(Fig. 6.8 |
c ). We know |
very little about the properties of recombination hot |
spots. Attempts to narrow them down to short specific DNA sequence elements have not yet succeeded in mammalian samples. However, we do know that recombination hot spots can,
themselves, be inherited alleles. This can lead to serious complications in trying to interpret genetic maps directly in terms of relative physical distances along the DNA.
Two other complications can lead to errors in the construction of genetic maps, and confuse the issue when these maps are compared directly with physical maps. If not properly identified and accounted for, both can lead to inconsistent evidence for gene order. Multiple crossovers can occur between a pair of DNAs strand during meiotic recombination. One schematic example of this is given in Figure 6.9, and the consequences for puta-
Figure 6.9 A more detailed view of meiotic recombination showing the association between pairs of homologous chromosomes after DNA synthesis, and the possibility of multiple crossovers before segregation.