
Genomics: The Science and Technology Behind the Human Genome Project. |
Charles R. Cantor, Cassandra L. Smith |
|
Copyright © 1999 John Wiley & Sons, Inc. |
|
ISBNs: 0-471-59908-5 (Hardback); 0-471-22056-6 (Electronic) |
2 |
A |
Genome |
Overview |
|
at |
the |
Level |
|
of |
Chromosomes |
BASIC PROPERTIES OF CHROMOSOMES
Chromosomes were first seen by light microscopy, and their name reflects the deep color they take on with a number of commonly used histological stains. In a cell a chromosome
consists |
of a single |
piece |
of DNA packaged |
with various accessory |
proteins. |
Chromosomes are the fundamental elements of inheritance, since it is they that are passed |
|||||
from cell to daughter cell, from parent to progeny. Indeed a cell that did not need to re- |
|||||
produce would not have to keep its DNA organized into specific large molecules. Some |
|||||
single-cell organisms, like the ciliate Tetrahymena, actually fragment a working copy of |
|||||
their DNA |
into gene-sized pieces for expression while maintaining an unbroken master |
||||
copy for reproductive purposes. |
|
|
|
||
BACTERIAL |
CHROMOSOMES |
|
|
|
|
Bacteria |
generally have |
a single |
chromosome. This |
is usually a circular DNA |
duplex. |
As shown in Figure 2.1, the chromosome has at least three functional elements. The repli- |
cation origin (ori) is the location of the start of DNA synthesis. The termination (ter) re-
gion provides a mechanism for stopping DNA synthesis of the two divergent replication |
|
|||||||
forks. Also present are |
|
par |
sequences which ensure that chromosomes are partitioned rel- |
|||||
atively uniformly between daughter cells. A description of a bacterial chromosome is |
||||||||
complicated by the fact that bacteria |
are continually |
replicating |
and |
transcribing |
their |
|||
DNA. |
|
|
|
|
|
|
|
|
In rapidly growing organisms, a round of replication is initiated |
before the previous |
|||||||
round is |
completed. Hence |
the |
number |
of copies of genomic DNA depends on how |
|
|||
rapidly the organism is growing and where in the genome one looks. Genes near the ori- |
|
|||||||
gin are often present at several times the copy number of genes near the terminus. In gen- |
||||||||
eral, this seems to have little effect on the bacterium. Rather, bacteria appear to take ad- |
||||||||
vantage of |
this fact. Genes |
whose |
products are required |
early in the |
replication cycle, |
or |
in large amounts (like the ribosomal RNAs and proteins), are located in the early replicated regions of the chromosomes. Although the bacterial chromosome is relatively toler-
ant of deletions (involving nonessential genes) and small insertions, many large rearrangements involving inversion or insertions are lethal. This prohibition appears to be related to conflicts that rise between convergent DNA replication forks and the transcrip-
tion machinery of highly expressed genes.
29
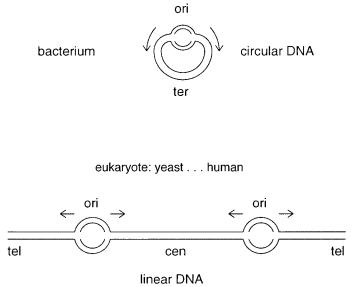
30 A GENOME OVERVIEW AT THE LEVEL OF CHROMOSOMES
Figure 2.1 Basic functional elements in chromosomes: ori (replication origin), tel (telomere), cen (centromere), ter (termination region). Little is known about eukaryotic termination regions.
Bacteria will frequently harbor additional DNA molecules smaller than their major chromosome. Some of these may be subject to stringent copy number control and to orderly partitioning, like the major chromosome. These low copy number plasmids use the
same DNA replication machinery as the |
chromosome, and they are present in a copy |
||
number that is equal to the chromosomal copy number. An example is the naturally oc- |
|||
curring F |
DNA of |
E. coli |
which represents about 2% of the DNA present in the cell. |
In some bacteria essential genes are located on two genetic elements. For instance, in |
|||
Rhodobacteria sphaeroides |
|
the genes encoding the ribosomal RNAs (rRNAs) are located |
|
on a 0.9 |
Mb chromosome, |
whereas the |
remainder of the genome is located on a 3 Mb |
chromosome. In Pseudomonas species, many genes that code for catabolic (degradative) enzymes are located on large extrachromosomal plasmids. Plasmids containing antibiotic resistance genes have been isolated from many species. In part, it is the rapid transfer of plasmids, sometimes even between different genera, that accounts for the rapid development of large populations of antibiotic resistant bacteria.
The control of replication of other, usually smaller plasmids, is more relaxed. These plasmids can have a very high intracellular copy number, and they are usually the focus
of recombinant DNA cloning experiments. These plasmids use a DNA replication mecha-
nism that is distinct from that used by the chromosomes. In fact selective inhibition of chromosomal replication machinery focuses the cell replication machinery on producing
more plasmid such that it is possible to increase the copy number of these plasmids to about 1000 copies per cell. Selection of growth conditions, which depend on genes carried by the plasmid, can also be used to increase or decrease its copy number. Some plasmids do not contain a par functioning region and are not partitioned in an orderly fashion
to daughter cells. This means that their inheritance is subject to statistical fluctuations that may lead to significant instabilities especially with low copy plasmids. Since the genome
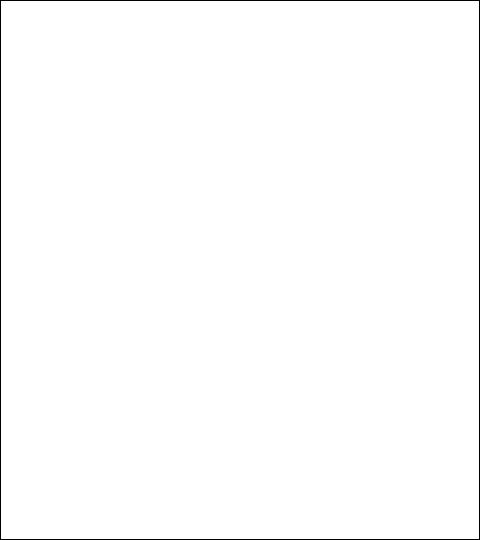
BACTERIAL CHROMOSOMES |
31 |
is defined as all of the DNA |
in a cell, plasmids and other extrachromosomal DNA ele- |
||
ments must be counted as part of it. |
|
||
Bacterial chromosomes contain bound protein molecules that are essential for normal |
|||
growth. Some of these promote an organization or packaging of DNA similar to that seen |
|||
in higher |
organisms. However, |
the proteins that appear to be responsible |
for packaging |
are present in such small amounts that can only interact with about 20% of the genomic |
|||
DNA. Furthermore the packaging does not seem to be as orderly or as stable as the pack- |
|||
aging of |
DNA in eukaryotic |
chromosomes. Bacterial chromosomal DNA is |
organized |
into topological constrained domains (Box 2.1) that average 75 kb in size. The way in which this occurs, and its functional consequences, are not yet understood in a rigorous
way.
BOX 2.1
TOPOLOGICAL PROPERTIES OF DNA
Because the two strands of DNA twist around a common axis, for unbent DNA, each
turn of the helix is equivalent to twisting one strand 360° around the other. Thus, when the DNA is circular, if one strand is imagined to be planar, the other is wrapped around it once for each helix turn. The two circular strands are thus linked topologically. They cannot be pulled apart except by cutting one of the strands. A single nick anywhere in either strand removes this topological constraint and allows strand separation. The topological linkage of the strands in circular DNA leads to a number of fascinating phenomena, and the interested reader is encouraged to look elsewhere for
detailed descriptions of how these are studied experimentally and how they are analyzed mathematically. (Cantor and Schimmel, 1980; Cozzarelli and Wang, 1990).
For the purpose of this book, the major thing the reader must bear in mind is that physical interactions between DNA strands can be blocked by topological constraints.
If a linear strand of DNA contacts a surface at two points, the region between these points is topologically equivalent to a circle that runs through the molecule and then through the surface. Hybridization of a complementary strand to this immobilized strand will require twisting the former around the latter. This may be difficult if the latter is close to the surface, and it will be impossible if the former is circular itself. Cells deal with the topological constraints of DNA double helices by having a se-
ries of enzymes that relax these constraints. Type I topoisomerases make a transient single-stranded nick in DNA, which allows one strand to rotate about the other at that point. Type II topoisomerases make a transient double-strand nick and pass an intact segment of the duplex through this nick. Though it is less obvious, this has the effect of allowing the strands to rotate 720° around each other. When DNA is replicated or transcribed, the double helix must be unwound ahead of and rewound behind the mov-
ing polymerases. Topoisomerases are recruited to enable these motions to occur.
|
In condensed chromatin, loops of 300 Å fiber are attached to a scaffold. Although |
|||||
the |
DNA of |
mammalian chromosomes is linear, |
these |
frequent attachment |
points |
|
make |
each |
constrained |
loop topologically into a |
circle. Thus topoisomerases are |
||
needed for |
the DNA in |
chromatin to function. |
Type |
II topoisomerases are |
a major |
component of the proteins that make up the chromosome scaffold.
32 A GENOME OVERVIEW AT THE LEVEL OF CHROMOSOMES
CHROMOSOMES OF EUKARYOTIC ORGANISMS
All higher organisms usually have linear chromosomal DNA molecules, although circles |
|
||||
can be produced under special circumstances. These molecules have a minimum of three |
|
||||
functional features, as shown in Figure 2.1. Telomeres are specialized |
structures |
at the |
|||
ends of the chromosome. These serve at |
least two functions. They provide a mechanism |
|
|||
by which the ends of the linear chromosomes can be replicated. They stabilize the ends. |
|||||
Normal double-strand DNA ends are very |
unstable in eukaryotic cells. Such ends could |
|
|||
be the result of DNA damage, such as that caused by X rays, and could be lethal events. |
|||||
Hence very efficient repair systems exist that rapidly ligate ends not containing telomeres |
|||||
together. If several DNAs are broken simultaneously in a single cell, the correct fragment |
|||||
pairs are unlikely to be reassembled, |
and one or more translocations will result. If the |
||||
ends are not repaired fast enough by ligation, they may invade duplex DNA in order to be |
|||||
repaired by recombination. The result, even for a single, original DNA break, is a re- |
|||||
arranged genome. |
|
|
|
|
|
|
Centromeres are DNA regions necessary for precise segregation of chromosomes to |
|
|||
daughter cells during cell division. They are the binding site for proteins that make up the |
|||||
kinetochore, which in turn serves as the attachment site for microtubules, the cellular or- |
|||||
ganelles that pull the chromosomes apart during cell division. |
|
|
|||
|
Another feature of eukaryotic chromosomes is replication origins. We know much less |
|
|||
about the detailed structural properties of eukaryotic origins than prokaryotic ones. What |
|||||
is clear from inspecting the pattern of DNA synthesis along chromosomes is that most |
|||||
chromosomes have many active replication origins. These do not necessarily all initiate at |
|||||
the same time, but a typical replicating chromosome will have many active origins. The |
|||||
presence of multiple replication origins allows for complete replication of entire human |
|||||
genome in only 8 hours. It is not known how these replication processes terminate. |
|
||||
CENTROMERES |
|
|
|
|
|
In |
the yeast, |
S. cerevisiae, |
the centromere has been defined by genetic and molecular ex- |
||
periments to reside in a small DNA region, about 100 bp in length. This region contains |
|||||
several A–T rich sequences that may be |
of key importance for function. The centromere |
||||
of |
S. cerevisiae |
is very different in size and characteristics than |
the centromeres of more |
||
advanced organisms, or even the yeast |
S. pombe. |
This is perhaps not too surprising in |
|||
view of the key role that the centromere plays in cell division. Unlike these other species, |
|||||
S. cerevisiae |
does not undergo symmetrical cell division. Instead, it buds and exports one |
||||
copy of each of its chromosomes to the daughter cell. In species that produce two nomi- |
|||||
nally identical daughter cells, centromeres appear to be composed mostly of DNA with |
|
||||
tandemly repeating sequences. The most striking feature of these repeats is that the num- |
|||||
ber of copies can vary widely. A small repeating sequence, (GGAAT) |
|
n , has recently been |
|||
found to be conserved across a wide range of species. This conservation suggests that the |
|||||
sequence may be a key functional element in centromeres. While not yet proved, physical |
|
||||
studies on this DNA sequence reveal it |
to have rather unusual helical properties that at |
||||
least make it an attractive candidate for a functional element. As shown in Figure 2.2, |
the |
G-rich strand of the repeat has a very stable helical structure of its own, although the detailed nature of this structure is not yet understood.
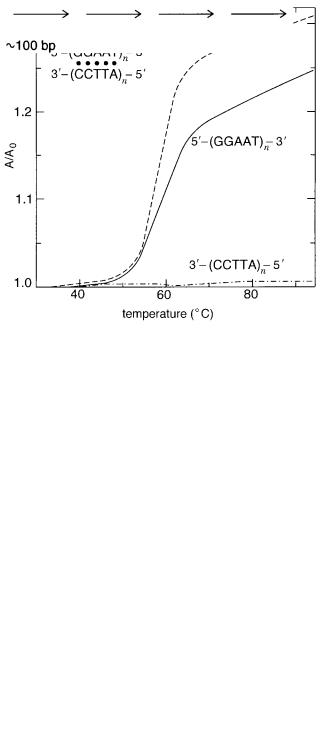
CENTROMERES 33
Figure 2.2 Evidence for the presence of some kind of unusual helical structure in a simple centromeric repeating sequence. The relative amount of absorbance of 260 nm UV light is measured as
a function of temperature. Shown are results for the two separated strands of a duplex, and the duplex itself. What is unusual is that one of the separated strands shows a change in absorbance that is almost as sharp and as large as the intact duplex. This indicates that it, alone, can form some sort of helical structure. (From Moyzis et al., 1988.)
The other repeats in the centromeres are much longer tandemly repeated structures. In
the human these were originally termed |
satellite DNA |
because they were originally seen |
as shoulders or side bands when genomic DNA fragments were fractionated by base com- |
|
|
position by equilibrium ultracentrifugation on CsCl gradients (see Chapter 5). For exam- |
||
ple, the alpha satellite of the african green |
monkey is a 171 base pair tandem |
repeat. |
Considerable effort has gone into determining the lengths and sequences of some of these satellites, and their organization on the chromosome. The results are complex. The repeats are often not perfect; they can be composed of blocks of different lengths or different orientation. The human alpha satellite, which is very similar in composition (65%
identity) to |
the african |
green monkey satellite, does |
not |
form |
a |
characteristic |
separate |
|||||||||
band during |
density |
ultracentrifugation. |
Thus |
the |
term |
satellite |
has |
evolved |
to |
now |
||||||
include |
tandemly |
repeated |
DNA |
sequences |
which |
may |
be |
of |
the |
same composition |
|
|
||||
of the |
majority |
of |
genome. |
An |
example |
of a satellite |
sequence |
is |
shown |
in Figure |
2.3. |
Figure 2.3 Example of a tandemly repeating DNA sequence in centromeric DNA.

34 |
A GENOME OVERVIEW AT THE LEVEL OF CHROMOSOMES |
|
|
|
||||||||
The implications of the specific sequences of the repeats, and their organization, for cen- |
||||||||||||
tromere function are still quite cloudy. |
|
|
|
|
|
|
|
|
|
|||
|
The size of centromeres, judged by the total length of their simple sequence blocks, |
|||||||||||
varies enormously among species, and even among the different chromosomes contained |
|
|
|
|||||||||
in one cell, without much indication that this is biologically important. Centromeres |
in |
S. |
||||||||||
pombe |
are only 10 |
4 to 10 5 |
bases in size, while those |
in the human can be several Mb. |
||||||||
Centromeres |
on different human chromosomes appear to be able |
to |
vary |
in |
size |
widely, |
|
|||||
and within the population there is great heterogeneity in the apparent size of some cen- |
||||||||||||
tromeres. It is as though nature, having found a good thing, doesn’t care how much of it |
||||||||||||
there is as long as it is more than a certain minimum. |
|
|
|
|
|
|
|
|
||||
TELOMERES |
|
|
|
|
|
|
|
|
|
|
|
|
Telomeres in almost all organisms with linear chromosomes are strikingly similar. They |
|
|||||||||||
consist of |
two components |
as shown in Figure |
2.4. At the very end of |
the |
chromosome |
is |
|
|||||
a long stretch of tandemly repeating sequence. In most organisms the telomere is domi- |
|
|||||||||||
nated by the hexanucleotide repeat (TTAGGG) |
|
|
|
n . The repeating pattern is not perfect; sev- |
||||||||
eral other sequences can be interspersed. In a few species the basic repeat is different, but |
||||||||||||
it always has the characteristic that one strand is T and G-rich. The |
G |
|
|
|
T rich strand is |
|||||||
longer than the complementary strand, and thus the ends of the chromosome have a pro- |
|
|||||||||||
truding 3 |
-end strand of some considerable length. This folds back on itself to make a sta- |
|||||||||||
ble helical structure. The best evidence suggests that this structure is four stranded, in |
||||||||||||
analogy to the four-strand helix made by aggregates of G itself. Pairs of telomeres might |
||||||||||||
have to associate in order to make this structure. Alternatively, it could be made by loop- |
||||||||||||
ing |
the 3 |
-end of one chromosome back on itself three times. The details not withstand- |
||||||||||
ing, these |
structures are apparently effective in protecting the ends of the |
chromosomes |
||||||||||
from attack by most common nucleases. |
|
|
|
|
|
|
|
|
|
|||
|
Next |
to the simple sequence telomeric repeats, most |
chromosomes |
have |
a |
series |
of |
|||||
more complex repeats. These frequently occur in blocks of a few thousand base pairs. |
||||||||||||
Within the blocks there may be some tandemly repeated sequence more complex than the |
|
|||||||||||
hexanucleotide telomeric repeat. The blocks themselves |
are |
of |
a number |
of |
different |
|||||||
types, and these are distributed in different ways on different chromosomes. Some unique |
|
|||||||||||
sequences, including genes, may occur between the repeating sequences. It is not clear if |
|
|||||||||||
any chromosome really has a unique telomere, or if this matters. Some |
researchers |
feel |
|
|||||||||
that the sub-telomeric repeats may play a role in positioning the ends of the chromosomes |
|
|||||||||||
at |
desired |
places within |
the nucleus. Whether and how this |
information |
might |
be |
coded |
|
||||
by the pattern of blocks on a particular chromosome remains |
to |
be determined. At |
least |
|
||||||||
some subtelomeric sequences vary widely from species to species. |
|
|
|
|
|
|
|
Figure 2.4 Structure of a typical telomere.
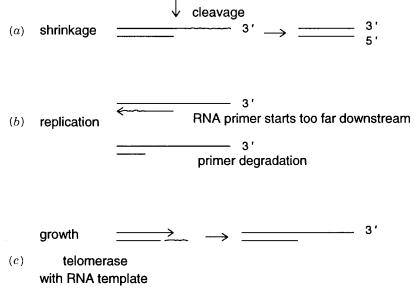
|
|
|
DYNAMIC BEHAVIOR OF TELOMERES |
35 |
|
DYNAMIC BEHAVIOR |
OF TELOMERES |
|
|
|
|
The actual length of the simple telomeric repeating DNA sequence is highly variable both |
|
||||
within species and between species. This appears to be a consequence, at least in part, of |
|
||||
the way in which telomeres are synthesized and broken down. Telomeres are not static |
|
||||
structures. If the |
growth |
of cells is monitored through successive generations, telomeres |
|
||
are observed to gradually shrink and then sometimes lengthen considerably. Nondividing |
|
|
|||
cells and some cancer cells appear to have abnormal telomere lengths. At least two mech- |
|
|
|||
anisms are known that can lead to telomere degradation. These are shown in Figure 2.5 |
|
a. |
|||
In one mechanism the single-strand extension is subject to some nuclease cleavage. This |
|
||||
shortens that strand. The alternate mechanism is based on the fact that the 5 |
|
-ended strand |
|||
must serve as a starting position for DNA replication. This presumably occurs by the gen- |
|
||||
eration of an RNA primer |
that is then extended inward by DNA |
replication. Because of |
|
||
the tandem nature of the repeated sequence, the primer can easily be displaced inward be- |
|
||||
fore synthesis continues. This will shorten the 5 |
-strand. Both of these mechanisms seem |
|
|||
likely to occur in practice. |
|
|
|
||
A totally different mechanism exists to synthesize telomeres and to lengthen existing |
|
||||
telomeres (Fig. 2.5 |
b ). The enzyme telomerase is present in all cells with telomeres. It is a |
|
|||
ribonucleoprotein. The RNA component is used as a template to |
direct the synthesis |
of |
|
||
the 3 -overhang of the telomere. Thus the telomere is lengthened by integral numbers of |
|
||||
repeat units. It is not known how the complex and subtle variations seen in this simple se- |
|
||||
quence arise in practice. Perhaps there is a family of telomerases with different templates. |
|
||||
More likely, some of the sequences are modified after synthesis, or the telomerase |
may |
|
|||
just be sloppy. |
|
|
|
|
|
Figure 2.5 Dynamics of telomeric simple repeating sequences. Mechanisms for telomere shrink-
age: (a)Nuclease cleavage; |
(b)downstream priming by telomerase; |
(c)mechanism of telomere |
growth. |
|
|
36 |
A GENOME OVERVIEW AT THE LEVEL OF CHROMOSOMES |
|
|
|
|
|
|
|||||||||||||||||
The total length of telomeric DNA in the human ranges from 10 to 30 kb. Because of |
|
|||||||||||||||||||||||
its heterogeneity, fragments of DNA cut from any particular telomere by restriction en- |
|
|
|
|||||||||||||||||||||
zymes have a broad size range and appear in gel electrophoretic size |
fractionations |
as |
|
|
||||||||||||||||||||
broad, fuzzy bands. This is sufficiently characteristic of telomere behavior to constitute |
|
|||||||||||||||||||||||
reasonable evidence that the band in question is telomeric. In mice, the length of telom- |
|
|
|
|||||||||||||||||||||
eres is much longer, typically 100 kb. We don’t know what this means. In general, the |
|
|||||||||||||||||||||||
message from both telomeres and centromeres is that eukaryotic cells apparently feel no |
|
|
|
|
||||||||||||||||||||
pressure to minimize the size of their genome or the sizes of these important functional |
|
|
||||||||||||||||||||||
elements. Unlike viruses, which must fit into small packages for cell escape and reinfec- |
|
|
|
|||||||||||||||||||||
tion, and bacterial cells which are under selection pressure |
in |
rich |
media |
to |
replicate |
as |
|
|
||||||||||||||||
fast as they can, eukaryotic cells are not mean and lean. |
|
|
|
|
|
|
|
|
|
|
|
|
|
|
|
|||||||||
CHROMATIN |
AND THE HIGHER-ORDER |
STRUCTURE |
|
|
|
|
|
|
|
|
|
|
|
|
|
|
|
|
|
|
||||
OF CHROMOSOMES |
|
|
|
|
|
|
|
|
|
|
|
|
|
|
|
|
|
|
|
|
|
|
||
A eukaryotic chromosome is only about half DNA by weight. The remainder is protein, |
|
|
|
|
|
|||||||||||||||||||
mostly histones. There are five related, very basic |
histone |
proteins |
that |
bind |
tightly |
to |
|
|||||||||||||||||
DNA. The rest is a complex mixture called, loosely, |
|
|
|
|
|
|
|
|
|
|
nonhistone |
chromosomal proteins. |
||||||||||||
This mixture consists of proteins needed to mediate successive higher-order packaging of |
|
|
|
|||||||||||||||||||||
the DNA, proteins needed for chromosome segregation, and proteins involved in gene ex- |
|
|
|
|
||||||||||||||||||||
pression |
and regulation. An enormous effort has gone into characterizing |
these |
proteins |
|
|
|
||||||||||||||||||
and the structures they form. Nevertheless, for the most part their role in higher-order |
|
|
||||||||||||||||||||||
structure or function is unknown. |
|
|
|
|
|
|
|
|
|
|
|
|
|
|
|
|
|
|
|
|
||||
At the lowest level of chromatin folding, 8 histones (two each of four types) assem- |
|
|||||||||||||||||||||||
ble into a globular core structure that binds about 140 bp of DNA |
forming |
it |
into |
a |
|
|||||||||||||||||||
coiled structure called the nucleosome (Fig. 2.6). Not only |
|
are |
nucleosomes |
very |
|
|||||||||||||||||||
similar |
in |
all |
organisms |
from |
yeast |
to |
humans |
but |
in |
addition |
|
the four core his- |
|
|||||||||||
tones are among the most evolutionarily conserved proteins known. Nucleosomes pack |
|
|
|
|
|
|||||||||||||||||||
together to form a filament that is 100 Å in diameter and is known by this name. The |
|
|||||||||||||||||||||||
details |
of the filament are different in different species |
because |
the |
lengths |
of |
the |
|
|||||||||||||||||
spacer DNA between the nucleosomes varies. |
In |
turn the |
100 |
Å |
|
filament |
is |
coiled |
|
|||||||||||||||
upon itself to make a thicker structure, called the 300 Å fiber. This appears to be |
||||||||||||||||||||||||
solenoidal in shape. Stretches of solenoid containing on average 50 to 100 kb of DNA |
|
|
||||||||||||||||||||||
are attached to a protein core. In condensed metaphase chromosomes, |
this |
core |
ap- |
|
|
|
||||||||||||||||||
pears as a central scaffold of the chromosome; it can be seen when the chromosome is |
|
|
|
|||||||||||||||||||||
largely |
stripped |
of other |
proteins and |
examined |
by |
electron |
microscopy (Fig. |
2.7). |
||||||||||||||||
A major component of this scaffold is the enzyme topoisomerase II (Box 2.1), which |
|
|||||||||||||||||||||||
probably serves a role analogous to DNA gyrase in |
|
|
|
|
|
|
|
|
|
|
|
|
E. |
coli |
of acting as a swivel to |
|||||||||
circumvent any topological problems caused by |
the |
interwound |
nature |
of |
DNA |
|
|
|
||||||||||||||||
strands. |
|
|
|
|
|
|
|
|
|
|
|
|
|
|
|
|
|
|
|
|
|
|
|
|
At other stages in the cell cycle, the scaffold proteins may actually attach to the nu- |
|
|||||||||||||||||||||||
clear envelope. The chromosome is then suspended from this envelope into the |
interior |
|
|
|
||||||||||||||||||||
of the nucleus. During mitosis, if this picture is correct, the chromosomes are then |
|
|||||||||||||||||||||||
essentially |
turned inside |
out, |
separated into |
daughters, |
and |
reinverted. |
The |
topologi- |
|
|||||||||||||||
cal domains created by DNA attachment to the scaffold at 50 to 100 kb intervals are |
|
|||||||||||||||||||||||
similar |
in size to those seen in bacteria, where they |
appear |
to |
|
be |
formed |
by |
much |
|
|||||||||||||||
simpler structures. |
|
|
|
|
|
|
|
|
|
|
|
|
|
|
|
|
|
|
|
|
|
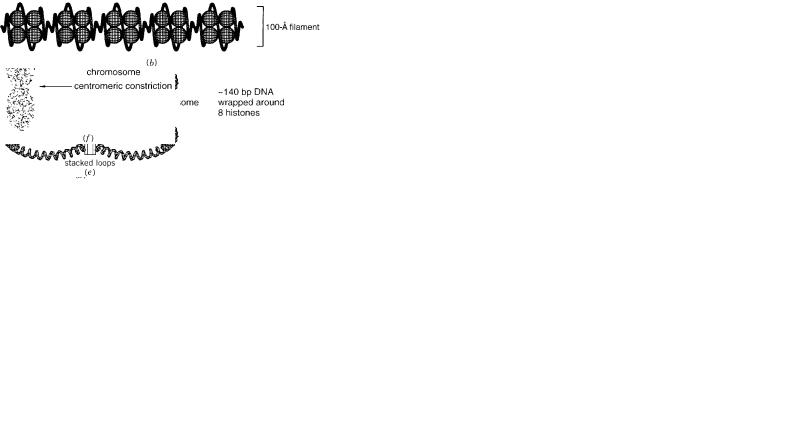
|
|
|
|
|
|
|
|
|
|
|
|
|
|
|
|
|
|
|
|
|
|
|
|
|
|
|
|
|
|
|
|
|
|
|
|
|
|
|
|
|
|
|
|
|
|
|
|
|
|
|
|
|
|
|
|
|
Figur |
e 2.6 Hierarchy |
of structural |
elements |
in |
chromatin |
and |
chromosomes: |
(a)The |
nucleosome; |
(b)a 100 Å fila- |
||||||||
ment; |
(c)a 300 Å solenoid. |
(d)chromosome |
loop |
anchored |
to |
a protein |
scaf fold; |
(e)successi |
ve |
loops |
stack |
ed |
along |
the |
||||
scaf fold; (f)the appearance |
of a condensed |
metaphase |
|
chromosome. |
|
The |
path of |
the scaf |
fold |
is |
unkno |
wn, |
b ut |
it is |
not |
|||
straight. |
|
|
|
|
|
|
|
|
|
|
|
|
|
|
|
|
|
|

38 A GENOME OVERVIEW AT THE LEVEL OF CHROMOSOMES
The most compact form of chromosomes occurs in metaphase. This is reasonable be- |
|
|||
cause it is desirable to pass chromosomes efficiently to daughter cells. Surely this is facil- |
|
|||
itated by having more compact structures than the unfolded objects seen in Figure 2.7. |
|
|||
Metaphase |
chromosomes appear to consist of stacks of packed 300 Å chromatin fiber |
|
||
loops. Their structures are quite well controlled. For example, they have a helical polarity |
|
|||
that is opposite |
in the two sister chromatids (the chromosome pairs about to separate in |
|
||
cell division). When the location of specific genes on metaphase chromosomes is exam- |
|
|||
ined (as described later), they appear to have a very fixed position within the morphologi- |
|
|||
cally visible structure of the chromosome. A characteristic feature of all metaphase chro- |
|
|||
mosomes is |
that |
the |
centromere region appears to be constricted. The reason for this is |
|
not known. |
Also |
for |
unknown reasons some eukaryotic organisms, such as the yeast |
S. |
cerevisiae |
|
do not have characteristic condensed metaphase chromosomes. |
|
|
The higher-order structure of chromatin and chromosomes poses an extraordinary |
|
|||
challenge for structural biologists because they are so complex and because these struc- |
|
|||
tures are so large. The effort needed to reveal the details of these structures may not be |
|
|||
worthwhile. It remains to be shown whether the details of much of the structure actually |
|
|||
matter for |
any |
particular biological function. One extreme possibility is that this is all |
|
|
cheap packaging; that most of it is swept away whenever the underlying DNA has to be |
|
|||
uncovered for function in gene expression or in recombination. We do not yet know if this |
|
|||
is the case, but our ability to manipulate DNAs and chromosomes has grown to the point |
|
|||
where it should soon be possible to test such notions explicitly. It would be far more ele- |
|
|||
gant and satisfying |
if we uncover sophisticated mechanisms that allow DNA packaging |
|
and unpackaging to be used to modulate DNA function.
Figure 2.7 Electron micrograph of a single mammalian chromosome, denatured to remove most of the protein and allow the DNA to expand. The X-shaped structure is the protein scaffold that de-
fines |
the shape of the condensed chromosome. The DNA appears as a barely resolvable mass of |
fiber |
covering almost the entire field. |