
Biomechanics Principles and Applications - Donald R. Peterson & Joseph D. Bronzino
.pdf

20-2 |
Biomechanics |
Nuclear |
CO2 |
energy |
|
|
O2 |
Heat |
|
|
Glucose |
Mechanical |
ATP |
|
|
work |
|
|
ADP |
FIGURE 20.1 Schematic of energy transformations leading to muscular mechanical work.
torque results in rotational acceleration. Static equilibrium requires that: |
|
F = 0 |
(20.1) |
T = 0 |
(20.2) |
where F is vectorial forces, N, and T is vectorial torques, N m.
Some sport activities, such as wrestling, weight lifting, and fencing, require stability, whereas other activities, including running, jumping, and diving, cannot be performed unless there is managed instability. Shifting body position allows for proper control. The mass of the body is distributed as in Table 20.1, and the center of mass is located at approximately 56% of a person’s height and midway from side-to- side and front-to-back. The center of mass can be made to shift by extending the limbs or by bending the torso.
TABLE 20.1 Fraction of Body
Weights for Various Parts of the Body
Body Part |
Fraction |
Head and neck |
0.07 |
Trunk |
0.43 |
Upper arms |
0.07 |
Forearms and hands |
0.06 |
Thighs |
0.23 |
Lower legs and feet |
0.14 |
|
1.00 |
|
|

Factors Affecting Mechanical Work in Humans |
20-3 |
dF
Fulcrum
Force
dW
Load
FIGURE 20.2 A class 3 lever is arranged with the applied force interposed between the fulcrum and the load. Most skeletal muscles are arranged in this fashion.
20.1.2 Muscular Movement
Mechanical movement results from contraction of muscles that are attached at each end to bones that can move relative to each other. The arrangement of this combination is commonly known as a class 3 lever (Figure 20.2), where one joint acts as the fulcrum (Figure 20.3), the other bone acts as the load, and the muscle provides the force interposed between fulcrum and load. This arrangement requires that the muscle force be greater than the load, sometimes by a very large amount, but the distance through which the muscle moves is made very small. These characteristics match muscle capabilities well (muscles can produce 7 × 105 N/m2, but cannot move far). Since the distance is made smaller, the speed of shortening
Biceps
Triceps
FIGURE 20.3 The biceps muscle of the arm is arranged as a class 3 lever. The load is located at the hand and the fulcrum at the elbow.
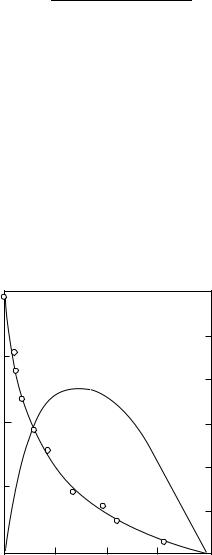

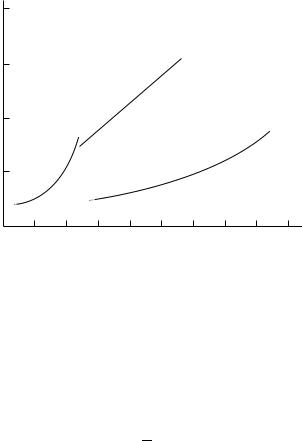
Factors Affecting Mechanical Work in Humans |
20-7 |
reason, it is difficult to determine exactly how much of the loss in capacity to exercise with age can really be attributed solely to the effects of aging. Nevertheless, the decline in cardiovascular or aerobic capacity with advanced aging is substantial. Aerobic capacity is assessed by measuring the maximal amount of oxygen that can be utilized during exercise and is known as VO2 max. Pollock et al. [1997] reported more than a 30% decline in VO2 max per decade of aging in a 20-year longitudinal study in those who did not maintain high levels of physical activity as they aged. This age-related decline was reduced substantially ( 5 to 8% per decade) when exercise training was maintained throughout aging. Thus, there is no doubt that maximal work capacity declines with advancing age. Although regular exercise (training) appears to reduce this decline substantially, it cannot completely prevent the detrimental effects of age on aerobic capacity.
Muscular strength (maximal force production) is maintained for a much longer time period throughout aging. Knee extensor strength, for example, does not become significantly reduced in men until they reach their 60s [Lynch et al., 1999]. These losses then occur at a rate of 12 to 14% per decade. Loss of muscle mass occurs at a rate of 6% per decade [Lynch et al., 1999]. There is some uncertainty about the magnitude of muscle power (product of force and velocity) loss with age [Martin et al., 2000], but it is believed to occur at a faster rate than strength [Skeleton et al., 1994]. There is also a loss of 8% per decade in force per unit of muscle mass (muscle quality) [Lynch et al., 1999], indicating that the loss of strength is more than can be accounted for by strength alone.
20.1.6 Effects of Exercise Training
For the purpose of this review, exercise training will be divided into two training modalities, aerobic exercise training and strength training. Aerobic exercise training consists of regular muscular activities that use large amounts of oxygen to produce energy and include exercises such as walking, jogging, swimming, and cycling. Strength training (ST) refers to the type of regular muscular activity that cause muscles to contract (e.g., isotonic or so-called concentric exercise) or attempt to contract (e.g., isometric or eccentric exercise) against resistance. Hence, this type of training is often called resistance or resistive training and it can be performed with weight plates, machines, or by just using one’s body weight for resistance, such as is done with certain kinds of calisthenics.
20.1.6.1 Aerobic Exercise Training
Many compensatory reactions allow the body to adapt to minor stresses, such as mild aerobic exercise, so that homeostasis (equilibrium) can be maintained. For example, the increased energy demands of aerobic exercise stimulate an increase in heart rate, respiration, blood flow, and many other cardiovascular and metabolic reactions that allow the body to maintain homeostasis. As the intensity of exercise increases it becomes more difficult for compensatory mechanisms to maintain homeostasis. After exceeding about 80% of an untrained person’s maximal exercise capacity homeostasis can no longer be maintained for more than a few minutes before exhaustion results.
Regular aerobic exercise (training) elevates the threshold level so that a single exercise session can be performed before disturbing and eventually losing homeostasis. It does this by elevating the maximal physiological capacity for homeostasis so that the same intensity of aerobic exercise may no longer disrupt homeostasis because it is a lower percentage of maximal capacity. In addition, training produces specific adaptations during submaximal exercise that permit greater and longer amounts of work before losing homeostasis [Hurley and Hagberg, 1998]. A good example of this is when blood lactate rises with increased intensity of exercise. Prior to training, blood lactate concentration rises substantially when the intensity of exercise exceeds about 75% of maximal oxygen consumption (VO2 max).
Following training, the same intensity of exercise results in a lower concentration of blood lactate, so exercise can now be performed at a higher fraction of the untrained VO2 max before blood lactate reaches the same level as before training. This adaptation is so profound that blood lactate levels are often only slightly above resting values after training when performing submaximal exercise (e.g., <65% of VO2 max). Thus, exercise training allows an individual to perform a much greater amount of work during exercise before homeostasis is disturbed to the point at which muscular exhaustion results.
20-8 |
Biomechanics |
20.1.6.2 Strength Training
Muscular strength declines with age [Lindle et al., 1997; Lynch et al., 1999; Roth et al., 2001], disease [Singh, 2002], and as a consequence of the administration of some medications [Singh, 2002] and can be increased substantially in a relatively short time period with ST [Hurley and Roth, 2000; Hurley and Kostek, 2001]. Muscle power can also be increased with ST, but the magnitude of increase depends on the movement velocity of training during the muscle-shortening phase of exercise. Typical training modalities of 2 sec during the shortening phase and 4 sec during the muscle-lengthening phase of movement result in 30% increase in strength [Lemmer et al., 2000] and 25% increase in power [Jozsi et al., 1999]. However, increases of almost 100% have been reported when fast-velocity training has been incorporated [Fielding et al., 2002]. Our group has reported increases in muscle mass of 12% with ST, within the first couple of months of training, based on measurements made by magnetic resonance imaging of the entire volume of the muscle being trained [Ivey et al., 2000]. The amount of force per unit of muscle volume also increases substantially with ST [Tracey et al., 1999]. We have shown that 3 decades of age-related strength loss and two decades of age-related muscle mass loss can be recovered/reversed within the first couple of months of ST [Ivey et al., 2000; Lemmer et al., 2000].
20.1.7 Effects of Gender
Cardiovascular fitness and muscular strength are substantially higher in men compared to women. The aerobic capacity in men is about 40 to 50% higher than women. In addition, upper body strength is 100% higher and lower body strength is 50% higher in men [Lynch et al., 1999]. However, these differences are diminished substantially when body composition is taken into consideration. For example, when VO2 max is expressed with reference to body mass (ml/kg of body weight/min) this difference narrows to 20% and to 10% when differences in muscle mass are taken into consideration. Differences in muscular strength between men and women are also narrowed when normalized for fat free mass [Lynch et al., 1999]. There does not appear to be any significant difference in responses to training between men and women when expressed on a relative basis (% change), but men appear to have greater muscle mass gains than women in response to ST when expressed in absolute terms [Ivey et al., 2000].
20.1.8 Effects of Genetics
We have observed great inter-individual variability in both the loss of muscle strength and mass with age, known as sarcopenia [Lindle et al., 1997; Lynch et al., 1999], as well as the gain in muscle strength and muscle mass with exercise training [Ivey et al., 2000; Lemmer et al., 2000]. For example, in a large number of ST studies performed in our laboratory, the increases in strength for individual subjects range from <5 to >100% and muscle mass changes range from no appreciable change to >25% increase. These ST-induced changes in strength and muscle mass are also highly variable in both young and older men and women. In this context, after only nine weeks of a highly standardized quadriceps ST program in a healthy and homogeneous group of young (20–30 yrs) and older (65–75 yrs) men and women, knee extension strength gains ranged from 9 to 45 kg in the young group and from 2 to 39 kg in the older group [Ivey et al., 2000]. These strength changes amounted to a 10–51% increase in the young group and a 5–59% increase in the older group. In this same study, the increases in MRI measured quadriceps muscle volume ranged from 19 to 344 cm3 in the older subjects and 60 to 481 cm3 in the young subjects. On a relative basis these increases ranged from <1 to 20% of the initial muscle volume of these subjects, further demonstrating the wide range of inter-individual changes in the trained muscle mass resulting from this highly standardized, short-term ST intervention. Others have observed similar large variations in strength and muscle mass changes with training [Buchner et al., 1993]. Collectively, these data strongly support the notion that genetic factors are involved in determining muscle strength and muscle mass responses to strength training.
The hypothesis that genetic factors may influence muscular strength is also supported by data from both animals [Biesiadecki et al., 1998] and humans [Reed et al., 1991]. Biesiadecki et al. [1998] observed a 1.5–5.2 fold divergence between the muscular strength of male rat strains with the lowestand
Factors Affecting Mechanical Work in Humans |
20-9 |
highest-strength levels, and Reed et al. [1991] observed significant genetic effects for absolute grip strength normalized for body weight in 127 identical and 130 fraternal twin pairs. Heredity accounted for 65% of the variance in grip strength, even after adjusting for the effects of weight, height, and age. Furthermore, Seeman et al. [1996] reported that genetic factors accounted for 60–80% of the inter-individual differences in lean body mass. More recently, Huygens et al. [2004] reported heritability of skeletal muscle mass of up to 90%. In addition, Thomis et al. [1998] found evidence for a significant role of heredity in strength responses to strength training. These responses were independent of the influence of genetic factors on baseline strength values. These data provide strong support that genetic factors may influence sarcopenia (i.e., alterations in strength and muscle mass with age) and strength and muscle mass response to strength training. However, none of these studies assessed the effects of specific candidate genes or candidate gene variations (polymorphisms) on strength or muscle mass response to training.
The heritability of muscle mass and strength indicate that specific genes contribute to differences in muscle phenotype. More importantly, specific polymorphisms could at least partly explain the inter-individual variability in sarcopenia and muscle response to ST. We recently explored the relationship of gene polymorphisms to muscle phenotypes in genes thought to have a plausible physiological connection to changes in strength and muscle mass. We identified several candidate gene polymorphisms that were associated with changes in strength or muscle mass with either age or ST. These included genes that code for ciliary neurotrophic factor, insulin-like growth factors I and II, growth and differentiation factor 8 (myostatin), Vitamin D, and androgen receptors. At the time of this writing, studies are ongoing to assess the role of these genes in determining the extent to which strength and muscle mass respond to aging and ST. Based on the substantial heritability of muscle phenotype, future research will likely find other gene variants that help explain the inter-individual variability in both sarcopenia and muscle adaptation in response to both aerobic and strength training. This information is likely to provide a basis for individualizing exercise prescriptions for the prevention and treatment of sarcopenia and help explain why some people make substantial adaptations to training, whereas others do not. Moreover, it will provide specific explanations from a mechanistic perspective as to how genetics can influence mechanical and biological work in humans.
References
Alexander, R.M. Walking and running. Am. Sci. 72: 348, 1984.
Biesiadecki, B.J., Brand, P.H., Metting, P.J., Koch, L.G., and Britton, S.L. Phenotypic variation in strength among eleven inbred strains of rats. Proc. Soc. Exp. Biol. Med. 219: 126–131, 1998.
Buchner, D.M., Cress, M.E., de Lateur, B.J., and Wagner, E.H. Variability in the effect of strength training on skeletal muscle strength in older adults. J. Gerontol. 7: 143–153, 1993.
Fiatarone-Singh, M.A. Exercise comes of age: rationale and recommendations for a geriatric exercise prescription. J. Gerontol.: Med. Sci. 57A: M262–M282, 2002.
Fielding, R.A., LeBrasseur, N.K., Cuoco, A., Bean, J., Mizer, K., and Fiatarone-Singh, M.A. High-velocity resistance training increases skeletal muscle peak power in older women. J. Am. Geriatr. Soc. 50: 655–662, 2002.
Goldman, R.F. Computer models in manual materials handling. In Drury, C.G. (Ed.), Safety in Manual Materials Handling, National Institute for Occupational Safety and Health (NIOSH), Cincinnati, OH, 110–116, 1978.
Hurley, B.F. and Hagberg, J.M. Optimizing health in older persons: aerobic or strength training? In Exercise and Sport Sciences Reviews, Vol. 26, Williams & Wilkins, Baltimore, MD, pp. 61–89, 1998.
Hurley, B.F. and Kostek, M.C. Exercise interventions for seniors. What training modality is best for health?
Orthop. Phys. Ther. Clin. N. Am. 10: 213–225, 2001.
Hurley, B.F. and Roth, S.M. Strength training in the elderly: effects on risk factors for age-related diseases. Sports Med. 30: 249–268, 2000.
Huygens, W., Thomis, M.A., Peeters, M.W., Vlietinck, R.F., and Beunen, G.P. Determinants and upper-limit heritabilities of skeletal muscle mass and strength. Can. J. Appl. Physiol. 29: 186–200, 2004.
20-10 |
Biomechanics |
Ivey, F.M., Tracy, B.L., Lemmer, J.T., Hurlbut, D.E., Martel, G.F., Roth, S.M., Fozard, J.L., Metter, E.J., and Hurley, B.F. The effects of age, gender and myostatin genotype on the hypertrophic response to heavy resistance strength training. J. Gerontol.: Med. Sci. 55A: M641–M648, 2000.
Johnson, A.T. Biomechanics and Exercise Physiology, John Wiley, New York, 1991.
Johnson, A.T., Benjamin, M.B., and Silverman, N. Oxygen consumption, heat production, and muscular efficiency during uphill and downhill walking. Appl. Ergon. 33: 485–491, 2002.
Jozsi, A.C., Campbell, W.W., Joseph, L., Davey, S.L., and Evans, W.J. Changes in power with resistance training in older and younger men and women. J. Gerontol. 54A: M591–M596, 1999.
Lemmer, J.T., Hurbut, D.E., Martel, G.F., Tracy, B.L., Ivey, F.M., Metter, E.J., Fozard, J.L., Fleg, J.L., and Hurley, B.F. Age and gender responses to strength training and detraining. Med. Sci. Sports Exer. 32: 1505–1512, 2000.
Lindle, R., Metter, E., Lynch, N., Fleg, J., Fozard, J., Tobin, J., Roy, T., and Hurley, B. Age and gender comparisons of muscle strength in 654 women and men aged 20–93. J. Appl. Physiol. 83: 1581–1587, 1997.
Lynch, N.A., Metter, E.J., Lindle, R.S., Fozard, J.L., Tobin, J.D., Roy, T.A., Fleg, J.L., and Hurley, B.F. Muscle quality I: age-associated differences in arm vs. leg muscle groups. J. Appl. Physiol. 86: 188–194, 1999.
Martin, J.C., Farrar, R.P., Wagner, B.M., and Spinduso, W.W. Maximal power across the lifespan. J. Gerontol.: Med. Sci. 55A: M311–M316, 2000.
McMahon, T.A. Muscles, Reflexes, and Locomotion, Princeton University Press, Princeton, NJ, 1984. Milsum, J.H. Biological Control Systems Analysis, McGraw-Hill, New York, 1966.
Pollock, M.L., Mengelkoch, L.F., Graves, J.S., Lowenthal, D.T., Limacher, M.C., Foster, C., and Wilmore, J.H. Twenty year follow-up of aerobic power and body composition of older track athletes. J. Appl. Physiol. 82: 1508–1516, 1997.
Reed, T., Babsitz, R., Selby, J., and Carmelli, D. Genetic influences and grip strength norms in the NHLBI twin study males aged 50–69. Ann. Hum. Biol. 18: 425–432, 1991.
Roth, S.M., Schrager, M.A., Ferrell, R.E., Reichman, Metter, S.E., Lynch, N.A., Lindle, R.S., and Hurley, B.F. Ciliary neurotrophic factor genotype is associated with muscular strength and quality in humans across the adult age span. J. Appl. Physiol. 90: 1205–1210, 2001.
Seeman, E., Hopper, J., Young, N., Formica, C., Goss, P., and Tsalamandris, C. Do genetic factors explain associations between muscle strength, lean mass, and bone density? A twin study. Am. J. Physiol. 270: E320–E327, 1996.
Skeleton, D.A., Greig, C.A., Davies, J.M., and Young, A. Power and related functional ability of healthy people aged 65–89 years. Age Aging 23: 371–377, 1994.
Thomis, M.A., Beunen, G.P., Maes, H.H., Blimkie, C.J., Leemputte, M.V., Claessens, A.L., Marchal, G., Willems, E., and Vlietinck, R.F. Strength training: importance of genetic factors. Med. Sci. Sports Exer. 30: 724–731, 1998.
Tracy, B.L., Ivey, F.M., Hurlbut, D., Martel, G.F., Lemmer, J.T., Siegel, E.L., Metter, E.J., Fozard, J.L., Fleg, J.L., and Hurley, B.F. Muscle quality II: effects of strength training in 65–75 year old men and women. J. Appl. Physiol. 86: 195–201, 1999.