
Color Atlas of Physiology 2003 thieme
.pdf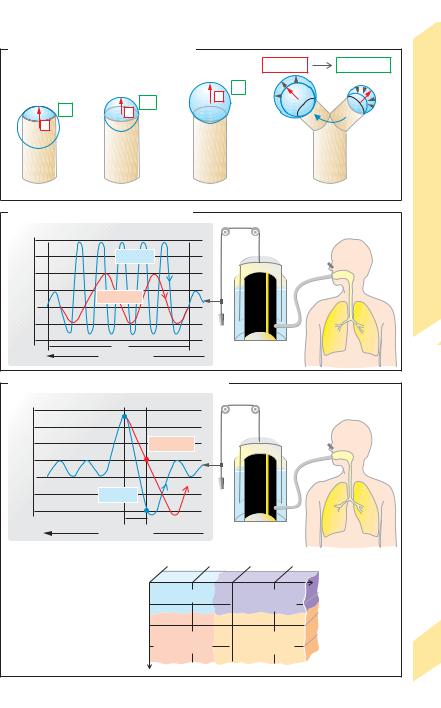
A. Surface tension (soap bubble model)
|
|
|
|
|
|
r1 > r2 |
P1 < |
P2 |
|
|
|
|
P |
|
r |
P |
|
r2 |
Tests |
|
|
|
|
|
|
||||
|
|
|
|
|
|
|
r1 |
|
|
|
P |
|
r |
|
|
P1 |
|
|
FunctionLung |
|
r |
|
|
|
|
|
|
P2 |
|
|
1 |
2 |
|
|
3 |
|
|
4 |
Tension, |
|
|
|
|
|
|
||||
B. Maximum breathing capacity (MBC) |
|
|
|
|
|
||||
|
Maximum respiratory depth and rate |
|
|
|
|
Surface |
|||
|
+2 |
Normal |
|
|
|
|
|
||
|
|
|
|
|
|
|
|
|
|
(L) |
+1 |
|
|
|
|
|
|
|
5.7 |
|
|
|
|
|
|
|
|
||
Volume |
0 |
Abnormal |
|
|
|
|
|
Plate |
|
|
|
|
|
|
|
|
|||
|
|
|
|
|
|
|
|
|
|
|
–1 |
10s |
|
|
|
Spirometer |
|
|
|
|
|
|
|
|
|
|
|
||
|
|
Paper feed |
|
|
|
|
|
|
|
C. Forced expired volume in first second (FEV1) |
|
|
|
|
|||||
|
+2 |
Maximum expiratory rate |
|
|
|
|
|
||
|
|
|
|
|
|
|
|
|
|
|
+1 |
|
|
Abnormal |
|
|
|
|
|
(L) |
|
|
|
|
|
|
|
|
|
Volume |
0 |
|
|
|
|
|
|
|
|
|
|
|
|
|
|
|
|
|
|
|
|
Normal |
|
|
|
|
|
|
|
|
–1 |
|
|
|
|
|
|
|
|
|
|
|
1s |
|
|
|
|
|
|
|
|
Paper feed |
|
|
|
|
|
|
|
1 Measurement |
|
|
|
|
|
|
|
|
|
|
|
|
|
1.0 |
0.8 |
0.6 |
0.4 |
|
|
|
|
|
1.0 |
|
|
|
|
Forced vital |
|
|
|
|
|
|
|
|
capacity (FVC) |
||
|
|
1 |
0.8 |
Normal |
|
Restrictive |
|
as fraction of norm |
|
|
|
FEV |
|
|
lung disease |
|
|
|
|
|
|
|
|
|
|
|
|
|
|
|
|
Relative |
0.6 |
|
|
|
|
|
|
|
|
|
|
|
|
|
|
|
|
|
|
|
|
Obstructive |
|
Combined |
|
|
|
|
2 |
|
0.4 |
lung disease |
lung disease |
|
|
119 |
|
|
|
|
|
|
|
|
|
||
|
Clinical significance |
|
|
|
|
|
|
||
|
|
|
|
|
|
|
|
Despopoulos, Color Atlas of Physiology © 2003 Thieme
All rights reserved. Usage subject to terms and conditions of license.

5 Respiration
120
Pulmonary Gas Exchange
Alveolar ventilation. Only the alveolar part (VA) of the tidal volume (VT) reaches the alveoli. The rest goes to dead space (VD). It follows that VA = VT – VD (L) (!p. 114). Multiplying these volumes by the respiratory rate (f in min. –.1) results. in the respective. ventilation,. . . i.e.,
VA, VE (or VT), and VD. Thus, VA = VE–VD (L ! min. – 1). Since VD is anatomically determined,
VD (= VD.! f) rises with f. If, at a given total ventilation (VE = VT ! f), the breathing becomes. more frequent (f ") yet more. shallow (VT #), VA will decrease because VD increases.
.
Example: At a VE of 8 L ! min– 1, a VD.of 0.15 L and a normal respiratory. rate f of 16 min-1 VA = 5.6 L ! min– 1 or 70%. of VE. When f is doubled and VT drops to onehalf,. VA drops to 3.2 L ! min– 1 or 40% of VT, although VE (8 L ! min– 1) remains unchanged.
Alveolar gas exchange can therefore decrease due to flat breathing and panting (e.g., due to a painful rib fracture) or artificial enlargement
of VD |
(!p. 134). |
. |
|
|
|
O2 consumption (VO2) is calculated as the |
difference between the inspired O2 volume/time |
|||||
. |
! FIO2, and |
the expired |
|
O2 volume/time |
|
(= VE |
. |
||||
. |
|
. |
|
|
|
(= VE |
!.FEO2. Therefore, VO2 |
= VE (FIO2 – FEO2). At |
|||
rest, VO2 !8 (0.21–0.17) = 0.32 L ! min– 1. |
|||||
|
|
|
|
|
. |
The eliminated CO2 volume (VCO2) is calcu- |
|||||
|
. |
|
|
|
|
lated as VT ! FECO2 (!0.26 L ! min – 1 at rest; FICO2 |
|||||
|
. |
. |
|
|
|
!0). VO2 and |
VCO2 increase about tenfold |
during. strenuous. physical work (!p. 74). The VCO2 to VO2 ratio is called the respiratory quotient (RQ), which depends on a person’s nutritional state. RQ ranges from 0.7 to 1.0 (!p. 228).
The exchange of gases between the alveoli and the blood occurs by diffusion, as described by Fick’s law of diffusion (!Eq. 1.7, p. 22,). The driving “force” for this diffusion is provided by the partial pressure differences between alveolar space and erythrocytes in pulmonary capillary blood (!A). The mean alveolar partial pressure of O2 (PAO2) is about 13.3 kPa
(100 mmHg) and that of CO2 (PACO2) is about 5.3 kPa (40 mmHg). The mean partial pres-
sures in the “venous” blood of the pulmonary artery are approx. 5.3 kPa (40 mmHg) for O2 (PVO2) and approx. 6.1 kPa (46 mmHg) for CO2 (PVCO2). Hence, the mean partial pressure difference between alveolus and capillary is
about 8 kPa (60 mmHg) for O2 and about 0.8 kPa (6 mmHg) for CO2, although regional variation occurs (!p. 122). PAO2 will rise when PACO2 falls (e.g., due to hyperventilation) and vice versa (!alveolar gas equation, p. 136).
O2 diffuses about 1–2 µm from alveolus to bloodstream (diffusion distance). Under normal resting conditions, the blood in the pulmonary capillary is in contact with the alveolus for about 0.75 s. This contact time (!A) is long enough for the blood to equilibrate with the partial pressure of alveolar gases. The capillary blood is then arterialized. PO2 and PCO2 in arterialized blood (PaO2 and PaCO2) are about the same as the corresponding mean alveolar pressures (PAO2 and PACO2). However, venous blood enters the arterialized blood through arteriovenous shunts in the lung and from bronchial and thebesian veins (!B). This extra-alveolar shunt as well as ventilation–per- fusion inequality (!p. 122) make the PaO2 decrease from 13.3 kPa (after alveolar passage) to about 12.0 kPa (90 mmHg) in the aorta (PaCO2 increases slightly; !A and p. 107).
The small pressure difference of about 0.8 kPa is large enough for alveolar CO2 exchange, since Krogh’s diffusion coefficient K for CO2 (KCO2 !2.5 ! 10–16 m2 ! s– 1 ! Pa– 1 in tissue) is 23 times larger than that for O2 (!p. 22). Thus, CO2 diffuses much more rapidly than O2. During physical work (high cardiac output), the contact time falls to a third of the resting value. If diffusion is impaired (see below), alveolar equilibration of O2 partial pressure is less likely to occur during physical exercise than at rest.
Impairment of alveolar gas exchange can occur for several reasons: (a) when the blood flow rate along the alveolar capillaries decreases (e.g., due to pulmonary infarction;
!B2), (b) if a diffusion barrier exists (e.g., due to a thickened alveolar wall, as in pulmonary edema; !B3), and (c) if alveolar ventilation is reduced (e.g., due to bronchial obstruction;
!B4 ). Cases B2 and B3 lead to an increase in functional dead space (!p. 114); cases B3 and
B4 lead to inadequate arterialization of the blood (alveolar shunt, i.e. non-arterialized blood mixing towards arterial blood). Gradual impairments of type B2 and B4 can occur even in healthy individuals (!p. 122).
Despopoulos, Color Atlas of Physiology © 2003 Thieme
All rights reserved. Usage subject to terms and conditions of license.

A. Alveolar gas exchange |
|
|
|
|
|
|
|
|
|
Alveolus |
|
|
|
|
PaCO2 |
= |
PaO2= |
Lung |
Pa (kPa) |
|
– |
5.33kPa |
13.33kPa |
|
|
||
Pv (kPa) |
(40mmHg) |
(100mmHg) |
capillary |
|
|
|
5.33 |
|
|
|
|
13.33 |
|
6.13 |
|
Erythrocyte |
|
5.33 |
Exchange |
|
|
|
|
|
|||
kPa |
mmHg |
|
mmHg kPa |
|
||
|
|
Gas |
||||
|
100 |
|
100 |
|
|
|
|
|
|
|
|
|
|
12 |
O2 |
|
12 |
|
Pulmonary |
|
10 |
80 |
|
80 |
10 |
|
|
|
|
|
|
|||
PO2 |
|
|
|
8 |
PCO2 |
|
8 |
60 |
|
60 |
|
||
6 |
CO2 |
40 |
6 |
|
5.8 |
|
|
|
|
Plate |
|||
|
40 |
|
|
|
||
|
|
ca. 0.75s |
|
|
||
|
|
|
|
|
|
|
B. Impairment of alveolar gas exchange |
|
|
|
|
||
|
Expiration |
CO2 |
Inspiration |
|
|
|
|
|
|
O2 |
|
|
|
1 |
|
|
Bronchial system |
|
|
|
Normal alveolar |
|
|
|
|
|
|
ventilation and perfusion |
|
|
|
|
|
|
|
|
|
|
Extra-alveolar shunt |
|
|
From pulmonary |
|
|
|
|
|
|
artery |
|
|
4 |
|
|
|
|
|
|
Non-ventilated alveolus |
|
||
2 |
|
|
|
|
|
|
Absent blood flow |
Functional |
|
Alveolar shunt |
|
|
|
|
|
|
|
|
||
|
|
|
|
|
|
|
|
dead space |
|
|
|
|
|
|
|
|
|
|
To |
|
3 |
|
|
|
|
pulmonary veins |
121 |
Diffusion barrier |
|
|
|
|
||
|
|
|
|
|
Despopoulos, Color Atlas of Physiology © 2003 Thieme
All rights reserved. Usage subject to terms and conditions of license.

|
Pulmonary Blood Flow, |
|
|
|
|||||||
|
|
Ventilation–Perfusion Ratio |
|
|
|
||||||
|
|
Neglecting the slight amount of blood that |
|||||||||
|
|
reaches the lungs via the bronchial arteries, |
|||||||||
|
|
|
|
|
|
|
. |
|
|
|
|
|
|
the mean pulmonary perfusion (Q), or blood |
|||||||||
|
|
flow to the lungs, is equal to the cardiac output |
|||||||||
|
|
(CO = 5–6 L/min). The pulmonary arterial pres- |
|||||||||
|
|
sure is about 25 mmHg in systole and 8 mmHg |
|||||||||
|
|
|
|
|
|
||||||
|
|
in diastole, with a mean (P) of about 15 mmHg. |
|||||||||
|
|
|
decreases to about 12 mmHg (Pprecap) in the |
||||||||
|
|
P |
|||||||||
|
|
precapillary region (up to the origin of the pul- |
|||||||||
|
|
monary capillaries) and about 8 mmHg in the |
|||||||||
|
|
postcapillary region (Ppostcap). These values |
|||||||||
|
|
apply to the areas of the lung located at the |
|||||||||
|
|
level of the pulmonary valve. |
|
|
|
||||||
Respiration |
Uneven distribution of blood flow within the lung |
||||||||||
(!A). Due to the additive effect of hydrostatic pres- |
|||||||||||
|
|||||||||||
|
|
sure (up to 12 mmHg), Pprecap increases in blood ves- |
|||||||||
|
|
sels below the pulmonary valves (near the base of the |
|||||||||
|
lung) when the chest is positioned upright. Near the |
||||||||||
5 |
|
apex of the lung, Pprecap decreases in vessels above |
|||||||||
|
the pulmonary valve (!A, zone 1). Under these con- |
||||||||||
|
|
ditions, Pprecap can even drop to subatmospheric |
|||||||||
|
levels, and the mean alveolar pressure (PA) is at- |
||||||||||
|
mospheric and can therefore cause extensive capil- |
||||||||||
|
lary compression (PA ! Pprecap ! Ppostcap; |
|
|
. |
|||||||
|
!A ). Q per |
||||||||||
|
unit of lung volume is therefore very small. In the |
||||||||||
|
|
central parts of the lung (!A, zone 2), luminal nar- |
|||||||||
|
rowing of capillaries can occur at their venous end, at |
||||||||||
|
least temporarily (Pprecap ! PA ! Ppostcap), while the |
||||||||||
|
|
area near the base of the lung (!A, zone 3) is con- |
|||||||||
|
|
tinuously supplied with blood (Pprecap ! Ppostcap ! PA). |
|||||||||
|
. |
|
|
|
|
|
|
|
|
||
|
|
Q per unit of lung volume therefore decreases from |
|||||||||
|
|
the apex of the lung to the base (!A, B, red line). |
|||||||||
|
|
|
Uneven |
distribution of |
alveolar |
ventilation. |
|||||
|
|
|
|
. |
|
|
|
|
|
|
|
|
|
Alveolar ventilation (VA) per unit of lung volume also |
|||||||||
|
increases from the apex to the base of the lungs due |
||||||||||
|
|
to the effects of gravity (!B , orange line), although |
|||||||||
|
|
|
|
. |
|
|
|
. . |
|||
|
not as much as Q. Therefore, the |
VA/Q ratio |
|||||||||
|
|
decreases from the apex to the base of the lung |
|||||||||
|
(!B, green curve and top scale). |
|
|
|
|||||||
|
. . |
|
|
|
. . |
|
|
|
|||
|
|
VA/Q imbalance. The mean VA/Q for the entire |
|||||||||
|
|
lung is 0.93 (!C2). This value is calculated |
|||||||||
|
|
|
|
|
|
|
|
. |
|
(ca. 5.6 |
|
|
|
from the mean alveolar ventilation VA |
|||||||||
|
|
|
|
|
|
|
. |
|
|
|
|
|
|
L/min) and total perfusion Q (ca. 6 L/min), |
|||||||||
|
|
which is equal to the cardiac output (CO). |
|||||||||
|
|
Under extreme conditions in which one part of |
|||||||||
|
|
|
|
|
|
|
. . |
|
|
|
|
|
|
the lung is not ventilated at all, VA/Q = 0 (!C1). |
|||||||||
|
|
In the other extreme in which blood flow is ab- |
|||||||||
|
. . |
|
|
|
|
|
|
|
|||
|
sent (VA/Q approaches infinity; !C3), fresh air |
||||||||||
122 |
|
conditions |
will prevail |
in the |
|
alveoli |
|||||
|
|
|
|
|
|
|
|
. . |
|||
|
|
(functional dead space; !p. 120). VA/Q can |
vary tremendously—theoretically, from 0 to ". In this case, the PAO2 will fluctuate between mixed venous PVO2 and PIO2 of (humidified). . fresh air (!D). In a healthy upright lung, VA/Q decreases greatly (from 3.3 to 0.63) from apex
to base at rest (!B, green line); PAO2 (PACO2) is therefore 17.6 (3.7) kPa in the “hyperventi-
lated” lung apex, 13.3 (5.3) kPa in the normally ventilated central zone, and 11.9 (5.6) kPa in the hypoventilated lung base. These changes are less pronounced. during physical exercise because Q also increases in zone 1 due to the corresponding. . increase in Pprecap.
VA/Q imbalance decreases the efficiency of the lungs for gas exchange. In spite of the high PAO2 at the apex of the lung (ca. 17.6 kPa; !D, right panel) and the fairly.normal mean PAO2 value, the relatively small Q fraction. of zone 1 contributes little to the total Q of the pulmonary veins. In this case, PaO2 # PAO2 and an alve- olar–arterial O2 difference (AaDO2) exists (normally about 1.3 kPa). When. a. total arteriovenous shunt is present (VA/Q = 0), even oxygen treatment will not help the patient, because it would not reach the pulmonary capillary bed (!C1).
Hypoxic vasoconstriction regulates alveolar perfusion. and. prevents the development of extreme VA/Q ratios. When the PAO2 decreases sharply, receptors in the alveoli emit local signals that trigger constriction of the supplying blood vessels. This throttles shunts in poorly ventilated or non-ventilated regions of the lung, thereby routing larger quantities of blood for .gas.exchange to more productive regions.
VA/Q imbalance can cause severe complications in many lung diseases. In shock lung. , for example, shunts can comprise 50% of Q. Lifethreatening lung failure can quickly develop if a concomitant pulmonary edema, alveolar diffusion barrier, or surfactant disorder exists (!p. 118).
Despopoulos, Color Atlas of Physiology © 2003 Thieme
All rights reserved. Usage subject to terms and conditions of license.

A. Regional blood flow in the lung (upright chest position)
Alveolus |
|
|
|
|
|
|
lung |
|
|
|
Zone 1 |
||
|
|
|
|
|
|
|
|
|
PA |
> Pprecap > Ppostcap |
|||
|
|
|
|
|
|
|
|
of |
|
||||
Pulmonary |
|
|
|
|
|
Pulmonary |
|
|
|
|
|
||
|
|
|
|
|
Level |
|
|
|
|
|
|||
artery |
|
|
|
|
|
|
vein |
|
|
|
Zone 2 |
||
|
|
|
|
|
|
|
|
|
|
|
Pprecap > PA > Ppostcap |
||
|
|
Lung |
|
|
|
|
|
|
|
|
Zone 3 |
||
|
|
|
|
|
|
|
|
|
|
|
|
||
|
|
|
|
|
|
|
|
|
Pprecap > Ppostcap > PA |
|
|
||
|
|
|
|
|
|
|
|
|
|
|
|
|
|
|
|
Pprecap |
PA |
Ppostcap |
|
|
|
|
Perfusion Q |
|
|||
|
|
|
|
|
|
|
|
|
|||||
B. Regional perfusion and |
|
|
|
|
|
|
· |
|
· |
||||
C. Effect of ventilation-perfusion ratio (VA/Q) |
|
||||||||||||
ventilation of lung |
|
on partial pressures in lung |
|
|
|
|
|||||||
|
|
|
|
|
Pressures in kPa |
Ambient air: PO2 = 20, PCO2 = 0 |
|||||||
0 |
|
VA/Q |
3 |
|
|
|
|
|
|
|
|
|
|
|
|
|
|
|
|
|
|
|
|
|
|
||
|
|
|
|
|
|
|
|
|
|
|
|||
Apex |
|
|
|
|
VA = 0 |
|
VA |
|
|
VA |
|
|
|
|
|
|
|
|
|
|
|
|
|
|
|
|
|
2 |
|
|
|
|
|
|
|
|
|
|
|
|
|
|
|
|
VA |
|
|
|
|
|
|
|
|
|
|
|
|
|
|
|
|
|
|
|
|
|
|
|
|
number |
|
|
|
Q |
PO2 |
= 5.33 |
PO2 =13.33 |
PO2 |
= 20 |
||||
|
|
|
|
PCO2 = 6.13 |
PCO2 = 5.33 |
PCO2 = |
0 |
||||||
|
|
|
Ventilation VA |
||||||||||
|
|
|
|
|
|
|
|
|
|
|
|
|
|
Rib |
|
|
Perfusion Q |
|
|
|
|
|
|
|
|
|
|
|
|
|
|
|
|
|
|
|
|
|
|
|
|
5 |
|
|
|
|
Q |
|
|
|
|
Q |
|
|
Q = 0 |
|
|
|
|
|
|
|
5.6 |
|
|
|
|||
Base |
|
|
|
|
VA |
= 0 |
VA |
= |
≈1 |
VA |
|
∞ |
|
|
|
|
|
|
|
6 |
|
|
|||||
0.5 |
|
1.0 |
1.5 |
Q |
Q |
|
Q |
|
|
||||
0 |
|
1 |
|
2 |
|
|
|
3 |
|
|
|||
|
|
(L/min per L lung) |
|
|
|
|
|
|
|||||
Q and |
VA |
Not ventilated |
Normal |
|
|
Not perfused |
|||||||
|
|
|
|
|
|
|
. . Plate 5.9 Pulmonary Blood Flow, VA–Q Ratio
D. Regional parameters of lung function
Ambient air
|
|
|
|
|
|
|
|
|
|
0.07 |
0.24 |
0.07 |
3.3 |
17.6 |
3.7 |
|
|
|
|
|
|
|
|
|
|
|
|
|
|
0.93 |
13.3 |
5.3 |
|
|
|
|
|
|
|
|
|
|
|
0.13 |
0.82 |
1.29 |
0.63 |
11.9 |
5.6 |
|
|
Mixed |
|
|
|
|
|
|
|
|
|
|
|
|
|
|
|
venous blood |
|
|
|
|
|
|
|
|
|
(L/min) |
|
(kPa) |
|
|
||
|
8 |
|
|
|
|
|
|
|
|
volume |
|
|
|
|||
(kPa) |
6 |
|
|
|
|
|
|
|
|
|
|
|
||||
4 |
|
|
|
|
|
|
|
|
|
|
|
|||||
2 |
|
|
|
|
|
|
|
|
|
lung |
|
|
|
|
|
|
CO |
2 |
|
|
|
|
|
|
|
|
of |
|
|
|
|
|
|
P |
|
|
|
|
|
|
|
|
Fraction |
|
|
|
|
|
|
|
|
|
|
|
|
|
|
|
|
|
|
· |
2 |
2 |
|
||
|
|
|
|
|
|
|
|
|
|
|
|
|
||||
0 |
|
|
|
|
|
|
|
|
A |
· |
/QA |
O |
CO |
|
||
|
6 |
8 |
10 |
12 |
14 |
16 |
18 |
20 |
· |
· |
PA |
PA |
|
|||
|
V |
Q |
V |
123 |
||||||||||||
|
|
|
|
PO2 (kPa) |
|
|
|
|
|
|
|
End-capillaries |
||||
|
|
|
|
|
|
|
|
|
|
|
|
|||||
|
|
|
|
|
|
|
|
|
|
|
|
(A, B, C, D after West et al.) |
|
Despopoulos, Color Atlas of Physiology © 2003 Thieme
All rights reserved. Usage subject to terms and conditions of license.

CO2 Transport in Blood
|
Carbon dioxide (CO2) is the end-product of |
|||
|
energy metabolism (!p. 228). CO2 produced |
|||
|
by cells of the body undergoes physical dissolu- |
|||
|
tion and diffuses into adjacent blood capillar- |
|||
|
ies. A small portion of CO2 in the blood remains |
|||
|
dissolved, while the rest is chemically bound in |
|||
|
form of HCO3– and carbamate residues of |
|||
|
hemoglobin (!A , lower panel, blue arrows; |
|||
|
!arteriovenous CO2 difference given in the |
|||
|
table). Circulating CO2-loaded blood reaches |
|||
|
the pulmonary capillaries via the right heart. |
|||
|
CO2 entering the pulmonary capillaries is re- |
|||
|
leased from the compounds (!A, red arrows), |
|||
|
diffuses into the alveoli, and is expired into the |
|||
Respiration |
atmosphere (!A and p. 106). |
|
||
The enzyme carbonic anhydrase (carbonate |
||||
dehydratase) catalyzes the reaction |
|
|||
HCO3– + H+ |
CO2 + H2O |
|
||
in erythrocytes (!A5, 7). Because it acceler- |
||||
ates the establishment of equilibrium, the |
||||
5 |
||||
short contact time (!1 s) between red blood |
||||
|
||||
|
cells and alveolus or peripheral tissue is suffi- |
|||
|
cient for the transformation CO2 |
HCO3–. |
||
|
CO2 diffusing from the peripheral cells (!A, |
|||
|
bottom panel: “Tissue”) increases PCO2 (approx. |
|||
|
5.3 kPa = 40 mmHg in arterial blood) to a mean |
|||
|
venous PCO2 of about 6.3 kPa = 47 mmHg. It also |
|||
|
increases the concentration of CO2 dissolved in |
|||
|
plasma. However, the major portion of the CO2 |
|||
|
diffuses into red blood cells, thereby increas- |
|||
|
ing their content of dissolved CO2. CO2 (+ H2O) |
|||
|
within the cells is converted to HCO3– (!A5, 2) |
|||
|
and hemoglobin carbamate (!A3). The HCO3– |
|||
|
concentration in erythrocytes therefore be- |
|||
|
comes higher than in plasma. As a result, about |
|||
|
three-quarters of the HCO3– ions exit the |
|||
|
erythrocytes by way of an HCO3–/Cl– anti- |
|||
|
porter. This anion exchange is also called Ham- |
|||
|
burger shift (!A4). |
|
||
|
H+ ions are liberated when CO2 in red cells |
|||
|
circulating in the periphery is converted to |
|||
|
HCO3– and hemoglobin (Hb) carbamate. |
|||
|
Bicarbonate formation: |
|
||
|
CO2 + H2O |
HCO3– + H+, |
[5.4] |
|
|
Hemoglobin carbamate formation: |
|||
|
Hb–NH2 + CO2 |
Hb–NH–COO– + H+. [5.5] |
||
|
Hemoglobin (Hb) is a key buffer for H+ ions in |
|||
124 |
the red cells (!A6; see also p. 140, “Non-bicar- |
|||
bonate buffers”). Since the removal of H+ ions |
in reactions 5.4 and 5.5 prevents the rapid establishment of equilibrium, large quantities of CO2 can be incorporated in HCO3– and Hb carbamate. Deoxygenated hemoglobin (Hb) can take up more H+ ions than oxygenated hemoglobin (Oxy-Hb) because Hb is a weaker acid (!A). This promotes CO2 uptake in the peripheral circulation (Haldane effect) because of the simultaneous liberation of O2 from erythrocytes, i.e. deoxygenation of Oxy-Hb to Hb.
In the pulmonary capillaries, these reactions proceed in the opposite direction (!A, top panel, red and black arrows). Since the PCO2 in alveoli is lower than in venous blood, CO2 diffuses into the alveoli, and reactions 5.4 and 5.5 proceed to the left. CO2 is released from HCO3– and Hb carbamate whereby H+ ions (released from Hb) are bound in both reactions (!A7, A8), and the direction of HCO3–/Cl– exchange reverses (!A9). Reoxygenation of Hb to Oxy-Hb in the lung promotes this process by increasing the supply of H+ ions (Haldane effect).
CO2 distribution in |
blood |
(mmol/L |
blood, |
|
1 mmol = 22.26 mL CO2) |
|
|
|
|
|
|
|
|
|
|
Dis- |
HCO3– Carba- |
Total |
|
|
solved |
|
mate |
|
|
CO2 |
|
|
|
|
|
|
|
|
Arterial blood: |
|
|
|
|
Plasma* |
0.7 |
13.2 |
0.1 |
14.0 |
Erythrocytes** |
0.5 |
6.5 |
1.1 |
8.1 |
Blood |
1.2 |
19.7 |
1.2 |
22.1 |
|
|
|
|
|
Mixed venous blood: |
|
|
|
|
Plasma* |
0.8 |
14.3 |
ca. 0.1 |
15.2 |
Erythrocytes** |
0.6 |
7.2 |
1.4 |
9.2 |
Blood |
1.4 |
21.5 |
1.5 |
24.4 |
|
|
|||
Arteriovenous CO2 difference in blood |
|
|||
|
0.2 |
1.8 |
0.3 |
2.3 |
Percentage of total arteriovenous difference |
||||
|
9% |
78% |
13% |
100% |
|
|
|
||
* Approx 0.55 L |
plasma/L blood; ** ca. |
0.45 L |
||
erythrocytes/L blood |
|
|
|
Despopoulos, Color Atlas of Physiology © 2003 Thieme
All rights reserved. Usage subject to terms and conditions of license.
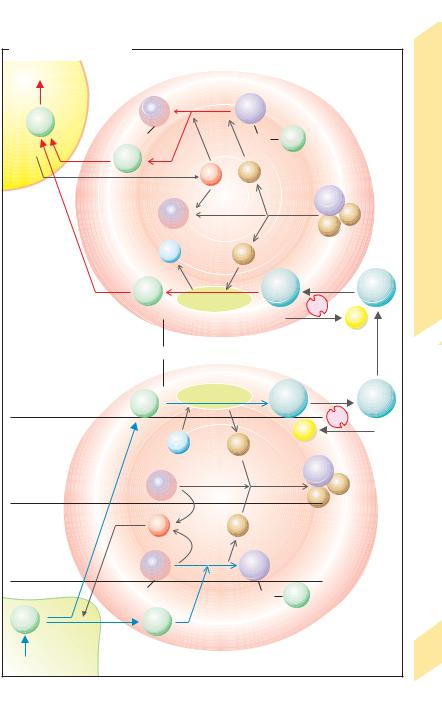
A. CO2 transport in blood |
|
|
|
|
|
|
|
|
||
Expelled from lung |
|
|
|
|
|
|
|
|
|
|
|
|
Oxy- |
|
8 |
Hb |
|
|
|
|
|
|
|
|
|
|
|
|
|
|
||
CO2 |
|
Hb |
|
|
|
|
|
|
|
|
|
|
|
|
|
|
|
|
|
|
|
|
|
NH2 |
|
|
NH |
COO– |
|
|
Blood |
|
|
|
CO2 |
|
|
|
|
|
|
|
|
Alveolus |
|
|
|
O2 |
H+ |
|
|
|
|
|
|
|
|
|
|
|
|
|
|
|
in |
|
|
Oxy- |
|
|
|
|
Hb |
|
-Transport |
|
|
|
|
|
|
|
H |
|
|||
|
|
Hb |
|
|
|
|
|
|||
|
|
|
|
|
|
H |
|
|||
|
|
|
|
|
|
|
|
|
||
|
|
|
|
|
|
|
|
|
|
|
In lung |
|
|
|
|
|
|
|
|
|
2 |
|
H2O |
|
|
H+ |
|
|
|
|
CO |
|
|
|
|
|
|
|
|
|
|||
|
|
|
|
7 |
|
|
|
9 |
|
5.10 |
|
|
|
|
|
HCO3– |
|
HCO3– |
|||
|
|
CO2 |
|
|
|
|
||||
|
|
Carbonic anhydrase |
|
|
|
|
Plate |
|||
|
|
|
|
|
|
|
||||
|
|
|
|
|
|
|
|
Cl– |
|
|
|
|
|
|
|
|
|
|
|
|
|
|
|
Erythrocyte |
|
|
|
|
|
In plasma |
|
|
|
|
|
|
|
|
|
|
|
|
|
In periphery |
|
|
5 |
|
|
|
|
|
|
|
|
|
|
|
|
|
|
|
|
|
|
Bicarbonate formation |
CO2 |
Carbonic anhydrase |
HCO3– |
|
HCO3– |
|
||||
|
|
|
|
|
|
|
|
|||
|
|
|
|
|
|
4 |
|
|
||
|
|
|
|
|
2 |
Cl– |
|
|
||
|
|
H2O |
|
|
|
|
||||
|
|
|
H+ |
|
|
|
|
|
||
|
|
Oxy- |
|
|
|
6 |
|
Hb |
|
|
Hemoglobin |
|
|
|
|
|
|
H |
|
|
|
|
Hb |
|
|
|
|
|
|
|
||
as buffer |
|
|
|
|
|
|
H |
|
|
|
|
|
|
|
|
|
|
|
|
||
|
|
O2 |
|
|
H+ |
|
|
|
|
|
Hemoglobin |
|
Oxy- |
|
|
Hb |
|
|
|
|
|
carbamate |
|
|
|
3 |
|
|
|
|
||
|
Hb |
|
|
|
|
|
|
|||
formation |
|
|
|
|
|
|
|
|
||
|
|
NH2 |
|
|
NH |
COO– |
|
|
|
|
CO2 |
|
CO2 |
|
|
Hemoglobin |
|
|
|
|
|
|
|
|
carbamate |
|
|
|
|
|||
1 |
Tissue |
|
|
|
|
|
|
|
|
125 |
|
|
|
|
|
|
|
|
|
||
Metabolism |
|
|
|
|
|
|
|
|
|
|
|
|
|
|
|
|
|
|
|
|
Despopoulos, Color Atlas of Physiology © 2003 Thieme
All rights reserved. Usage subject to terms and conditions of license.

5 Respiration
126
CO2 Binding in Blood, CO2 in CSF
The total carbon dioxide concentration (= chemically bound “CO2” + dissolved CO2) of mixed venous blood is about 24–25 mmol/L; that of arterial blood is roughly 22–23 mmol/L. Nearly 90% of this is present as HCO3– (!A, right panel, and table on p. 124). The partial pressure of CO2 (PCO2) is the chief factor that determines the CO2 content of blood. The CO2 dissociation curve illustrates how the total CO2 concentration depends on PCO2 (!A).
The concentration of dissolved CO2, [CO2], in plasma is directly proportional to the PCO2 in plasma and can be calculated as follows:
[CO2] = αCO2 ! PCO2 (mmol/L plasma |
|
or mL/L plasma), |
[5.6] |
where αCO2 is the (Bunsen) solubility coefficient for CO2. At 37 !C,
αCO2 = 0.225 mmol ! L–1 ! kPa–1,
After converting the amount of CO2 into volume CO2 (mL = mmol ! 22.26), this yields
αCO2 = 5 mL ! L–1 ! kPa–1.
The curve for dissolved CO2 is therefore linear (!A, green line).
Since the buffering and carbamate formation capacities of hemoglobin are limited, the relation between bound “CO2” and PCO2 is curvilinear. The dissociation curve for total CO2 is calculated from the sum of dissolved and bound CO2 (!A, red and violet lines).
CO2 binding with hemoglobin depends on the degree of oxygen saturation (SO2) of hemoglobin. Blood completely saturated with O2 is not able to bind as much CO2 as O2-free blood at equal PCO2 levels (!A, red and violet lines). When venous blood in the lungs is loaded with O2, the buffer capacity of hemoglobin and, consequently, the levels of chemical CO2 binding decrease due to the Haldane effect (!p. 124). Venous blood is never completely void of O2, but is always O2-satu- rated to a certain degree, depending on the degree of O2 extraction (!p. 130) of the organ in question. The SO2 of mixed venous blood is about 0.75. The CO2 dissociation curve for SO2 = 0.75 therefore lies between those for SO2 = 0.00 and 1.00 (!A, dotted line). In arterial blood,
PCO2 !5.33 kPa and SO2 !0.97 (!A, point a). In mixed venous blood, PCO2 !6.27 kPa and SO2
!0.75 (!A, point V). The normal range of CO2
dissociation is determined by connecting these two points by a line called “physiologic CO2 dissociation curve.”
The concentration ratio of HCO3– to dissolved CO2 in plasma and red blood cells differs (about 20 : 1 and 12 : 1, respectively). This reflects the difference in the pH of plasma (7.4) and erythrocytes (ca. 7.2) (!p. 138ff.).
CO2 in Cerebrospinal Fluid
Unlike HCO3– and H+, CO2 can cross the bloodcerebrospinal fluid (CSF) barrier with relative
ease (!B1 and p. 310). The PCO2 in CSF therefore adapts quickly to acute changes in the PCO2
in blood. CO2-related (respiratory) pH changes in the body can be buffered by non-bicarbonate buffers (NBBs) only (!p. 144). Since the concentration of non-bicarbonate buffers in CSF is very low, an acute rise in PCO2 (respiratory acidosis; !p. 144) leads to a relatively sharp decrease in the pH of CSF (!B1, pH""). This decrease is registered by central chemosensors (or chemoreceptors) that adjust respiratory activity accordingly (!p. 132). (In this book, sensory receptors are called sensors in order to distinguish them from hormone and transmitter receptors.)
The concentration of non-bicarbonate buffers in blood (hemoglobin, plasma proteins) is high. When the CO2 concentration increases, the liberated H+ ions are therefore effectively buffered in the blood. The actual HCO3– concentration in blood then rises relatively slowly, to ultimately become higher than in the CSF. As a result, HCO3– diffuses (relatively slowly) into the CSF (!B2), resulting in a renewed increase in the pH of the CSF because the HCO3–/CO2 ratio increases (!p. 140). This, in turn, leads to a reduction in respiratory activity (via central chemosensors), a process enhanced by renal compensation, i.e., a pH increase through HCO3– retention (!p. 144). By this mechanism, the body ultimately adapts to chronic elevation in PCO2— i.e., a chronically elevated PCO2 will no longer represent a respiratory drive (cf. p. 132).
Despopoulos, Color Atlas of Physiology © 2003 Thieme
All rights reserved. Usage subject to terms and conditions of license.
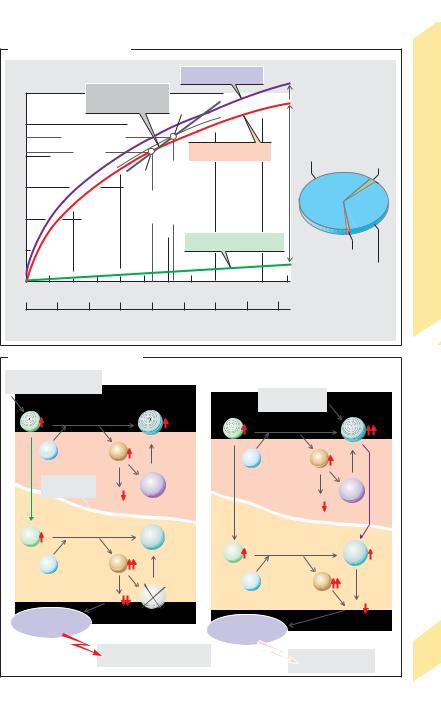
A. CO2 dissociation curve |
|
|
|
|
|
|
|
|
|||
CO2 concentration |
|
|
|
O2 saturation = 0.00 |
|
|
|
||||
of blood (mmol/L) |
|
|
|
|
|
|
|||||
|
|
|
|
|
|
|
|
|
|||
30 |
|
|
|
|
- |
|
|
|
|
|
|
|
|
Normal range: a–v |
- |
|
|
|
|
CSF |
|||
|
|
|
|
|
|
v |
|
|
|
|
|
25 |
|
|
|
|
|
|
|
|
|
|
|
|
|
|
|
|
|
|
|
Total CO2 in blood |
|
in |
|
|
|
mixed venous |
|
|
|
|
|
|
|||
|
|
|
|
|
|
|
|
2 |
|||
|
|
|
|
|
|
|
(=100%) |
|
|||
|
|
|
|
|
|
|
|
CO |
|||
|
|
|
|
|
|
|
|
|
|
||
20 |
|
arterial |
|
|
|
O2 saturation = 1.00 |
Plasma-HCO3– |
|
|||
|
|
|
|
|
Binding in Blood, |
||||||
|
|
|
|
|
CO2 |
||||||
|
|
|
|
|
|
|
|
|
2 |
||
|
|
|
|
a |
|
|
|
|
|
|
|
15 |
|
|
|
|
|
|
|
|
60% |
|
|
|
|
|
|
|
|
|
|
|
|
||
|
|
|
|
|
|
|
|
|
29% |
||
10 |
|
|
|
arterial |
venousmixed |
|
|
|
BoundCO |
|
|
|
|
|
|
|
|
|
|
||||
|
|
|
|
|
|
|
Dissolved CO2 |
|
|
2 |
|
5 |
|
|
|
|
|
|
Carbamate |
|
CO |
||
|
|
|
|
|
|
|
|
|
|||
|
|
|
|
|
|
|
|
|
RBC-HCO3– |
5.11 |
|
0 |
|
|
|
|
|
|
|
|
|
|
|
0 |
|
2 |
4 |
|
6 |
|
8 |
10 |
P |
|
|
|
|
kPa |
|
Plate |
|||||||
|
|
|
|
|
|
|
|
|
CO2 |
|
|
0 |
10 |
20 |
30 |
40 |
|
50 |
60 |
70 |
80 |
|
|
|
|
|
|||||||||
|
|
|
|
|
|
mmHg |
|
|
|
|
|
B. Effect of CO2 on pH of CSF |
|
|
|
|
||
Example: |
|
1 Acute |
|
2 Chronic |
||
Respiratory acidosis |
|
|
|
|||
|
|
|
|
|
Renal |
|
|
|
|
|
|
compensation |
|
CO2 |
|
|
HCO3– |
CO2 |
HCO3– |
|
|
|
|
|
|
||
Rapid diffusion |
H2O |
H+ |
Blood |
H2O |
H+ |
|
|
|
|
||||
Blood-CSF |
|
NBB |
|
|
diffusion |
|
barrier |
pH |
|
NBB |
|||
|
|
|||||
|
|
|
|
|||
|
|
|
|
pH |
||
|
|
|
|
|
|
Slow |
CO2 |
|
|
HCO3– |
|
|
|
|
|
H+ |
|
CO2 |
HCO3– |
|
|
H2O |
CSF |
|
|
|
|
|
|
|
|
H2O |
H+ |
|
|
|
pH |
NBB |
|
pH ( |
) |
|
|
|
|
|||
Central |
|
|
|
|||
|
|
Central |
|
|
||
chemoreceptors |
|
|
|
|
||
|
|
|
|
chemoreceptors |
|
|
|
|
Strong signal for |
|
Weak signal |
127 |
|
|
|
respiratory regulation |
|
|||
|
|
|
(adaptation) |
|
||
|
|
|
|
|
|
Despopoulos, Color Atlas of Physiology © 2003 Thieme
All rights reserved. Usage subject to terms and conditions of license.
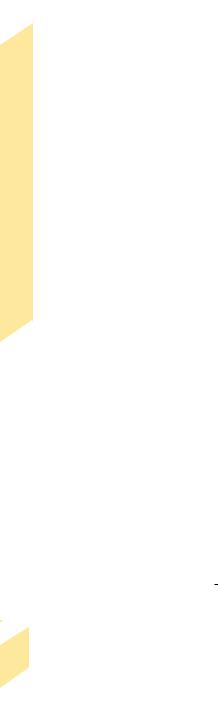
5 Respiration
128
Binding and Transport of O2 in Blood
Hemoglobin (Hb) is the O2-carrying protein of red blood cells (RBCs) (mol. mass: 64 500 Da). Hb is also involved in CO2 transport and is an important blood pH buffer (!pp. 124 and 138ff.). Hb is a tetramer with 4 subunits (adults: 98%: 2α + 2" = HbA; 2% 2α + 2δ = HbA2), each with its own heme group. Heme consists of porphyrin and Fe(II). Each of the four Fe(II) atoms (each linked with one histidine residue of Hb) binds reversibly with an O2 molecule. This is referred to as oxygenation (not oxidation) of Hb to oxyhemoglobin (OxyHb). The amount of O2 which combines with Hb depends on the partial pressure of O2 (PO2): oxygen dissociation curve (!A, red line). The curve has a sigmoid shape, because initially bound O2 molecules change the conformation of the Hb tetramer (positive cooperativity) and thereby increase hemoglobin-O2 affinity.
When fully saturated with O2, 1 mol of tetrameric Hb combines with 4 mol O2, i.e., 64 500 g of Hb combine with 4 !22.4 L of O2. Thus, 1 g Hb can theoretically transport 1.39 mL O2, or 1.35 mL in vivo (Hüfner number). The total Hb concentration of the blood
([Hb]total) is a mean 150 g/L (!p. 88), corresponding to a maximum O2 concentration of
9.1 mmol/L or an O2 fraction of 0.203 L O2/L blood. This oxygen-carrying capacity is a func-
tion of [Hb]total (!A, yellow and purple curves as compared to the red curve).
The O2 content of blood is virtually equivalent to the amount of O2 bound by Hb since only 1.4% of O2 in blood is dissolved at a PO2 of 13.3 kPa (!A, orange line). The solubility coefficient (αO2), which is 10 µmol ! [L of plasma]– 1 ! kPa– 1, is 22 times smaller
than αCO2 (!p. 126).
Oxygen saturation (SO2) is the fraction of Oxy-Hb relative to [Hb]total, or the ratio of actual O2 concentration/ O2-carrying capacity. At normal PO2 in arterial blood (e.g., PaO2 = 12.6 kPa or 95 mmHg), SO2 will reach a saturation plateau at approx. 0.97, while SO2 will still amount to 0.73 in mixed venous blood (PVO2 = 5.33 kPa or 40 mmHg). The venous SO2 values in different organs can vary greatly (!p. 130).
O2 dissociation is independent of total Hb if plotted as a function of SO2 (!B). Changes in O2 affinity to Hb can then be easily identified as shifting of the O2 dissociation curve. A shift to
the right signifies an affinity decrease, and a shift to the left signifies an affinity increase, resulting in flattening and steepening, respectively, of the initial part of the curve. Shifts to the left are caused by increases in pH (with or without a PCO2 decrease) and/or decreases in PCO2, temperature and 2,3-bisphosphoglyc- erate (BPG; normally 1 mol/mol Hb tetramer). Shifts to the right occur due to decreases in pH and/or increases in PCO2, temperature and 2,3- BPG (!B). The half-saturation pressure (P0.5 or
P50) of O2 (!B, dotted lines) is the PO2 at which SO2 is 0.5 or 50%. The P0.5, which is normally 3.6 kPa or 27 mmHg, is a measure of shifting to
the right (P0.5") or left (P0.5#). Displacement of the O2 dissociation curve due to changes in pH and PCO2 is called the Bohr effect. A shift to the right means that, in the periphery (pH#, PCO2"), larger quantities of O2 can be absorbed from the blood without decreasing the PO2, which is the driving force for O2 diffusion (!B, broken lines). A higher affinity for O2 is then re-established in the pulmonary capillaries (pH", PCO2#). A shift to the left is useful when the PAO2 is decreased (e.g., in altitude hypoxia), a situation where arterial SO2 lies to the left of the SO2 plateau.
Myoglobin is an Fe(II)-containing muscle protein that serves as a short-term storage molecule for O2 (!p. 72). As it is monomeric (no positive cooperativity), its O2 dissociation curve at low PO2 is much steeper than that of HbA (!C). Since the O2 dissociation curve of fetal Hb (2α + 2γ = HbF) is also steeper, SO2 values of 45 to 70% can be reached in the fetal umbilical vein despite the low PO2 (3–4 kPa or 22–30 mmHg) of maternal placental blood. This is sufficient, because the fetal [Hb]total is 180 g/L. The carbon monoxide (CO) dissociation curve is extremely steep. Therefore, even tiny amounts of CO in the respiratory air will dissociate O2 from Hb. This can result in carbon monoxide poisoning (!C). Methemoglobin, Met-Hb (normally 1% of Hb), is formed from Hb by oxidation of Fe(II) to Fe(III) either spontaneously or via exogenous oxidants. Met-Hb cannot combine with O2 (!C). Methemoglobin reductase reduces Fe(III) of Met-Hb back to Fe(II); deficiencies of this enzyme can cause methemoglobinemia, resulting in neonatal anoxia.
Despopoulos, Color Atlas of Physiology © 2003 Thieme
All rights reserved. Usage subject to terms and conditions of license.