
Enzymes (Second Edition)
.pdf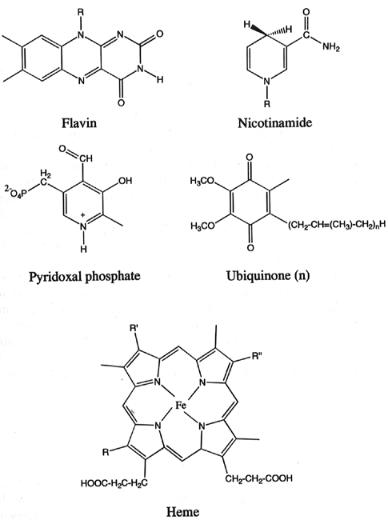
70 STRUCTURAL COMPONENTS OF ENZYMES
Figure 3.19 Examples of some common cofactors found in enzymes.
Cofactors fulfill a broad range of reactions in enzymes. One of the more common roles of enzyme cofactors is to provide a locus for oxidation/reduction (redox) chemistry at the active site. An illustrative example of this is the chemistry of flavin cofactors.
Flavins (from the Latin word flavius, meaning yellow) are bright yellow ( 450 nm) cofactors common to oxidoreductases, dehydrogenases, and electron transfer proteins. The main structural feature of the flavin cofactor is the highly conjugated isoalloxazine ring system (Figure 3.19). Oxidized flavins readily undergo reversible two-electron reduction to 1,5-dihydroflavin, and
SUMMARY 71
thus can act as electron sinks during redox reactions within the enzyme active site. For example, a number of dehydrogenases use flavin cofactors to accept two electrons during catalytic oxidation of NADH (another common enzyme cofactor; see Nicotinamide in Figure 3.19). Alternatively, flavins can undergo discrete one-electron reduction to form a semiquinone radical; this can be further reduced by a second one-electron reduction reaction to yield the fully reduced cofactor. Through this chemistry, flavin cofactors can participate in one-electron oxidations, such as those carried out during respiratory electron transfer in mitochondria. There are actually two stable forms of the flavin semiquinone that interconvert, depending on pH (Figure 3.20) The blue neutral semiquinone occurs at neutral and acidic pH, while the red anionic semiquinone occurs above pH 8.4. Both forms can be stabilized and observed in certain enzymatic reactions.
Additional chemical versatility is demonstrated by flavin cofactors in their ability to form covalent adducts with substrate during redox reactions. The oxidation of dithiols to disulfides by the active site flavin of glutathione reductase is an example of this. Here the thiolate anion adds to the C4a carbon of the isoalloxazine ring system (Figure 3.21A). Likewise, in a number of flavoenzyme oxidases, catalytic reoxidation of reduced flavin by molecular oxygen proceeds with formation of a transient C4a peroxide intermediate (Figure 3.21B).
A variety of other cofactors participate in the catalytic chemistry of the enzyme active site. Some additional examples of these are listed in Table 3.4. This list is, however, far from comprehensive; rather it gives just a hint of the breadth of structures and reactivities provided to enzymes by various cofactors. The texts by Dixon and Webb (1979) Walsh (1979), and Dugas and Penney (1981) give more comprehensive treatments of enzyme cofactors and the chemical reactions they perform.
3.8 SUMMARY
In this chapter we have seen the diversity of chemical reactivities that are imparted to enzymes by the structures of the amino acid side chains. We have described how these amino acids can be linked together to form a polypeptide chain, and how these chains fold into regular patterns of secondary and tertiary structure. The folding of an enzyme into its correct tertiary structure provides a means of establishing the binding pockets for substrate ligands and presents, within these binding pockets, the chemically reactive groups required for catalysis. The active site of the enzyme is defined by these reactive groups, and by the overall topology of the binding pocket. We have seen that the chemically reactive groups used to convert substrate to product molecules are recruited by enzymes, not only from the amino acids that make up the protein, but from cofactor molecules as well; these cofactors are critical components of the biologically active enzyme molecule. In Chapter 6, we shall see how the
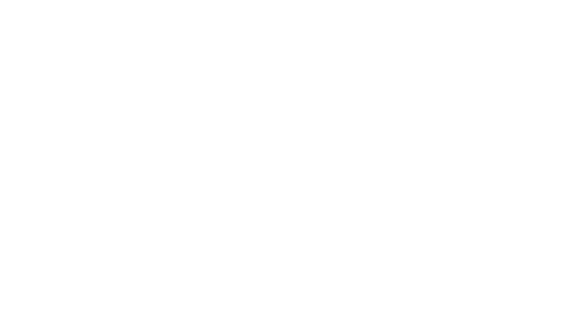
ENZYMES OF COMPONENTS STRUCTURAL 72
Figure 3.20 Structures of the flavin cofactor in its various oxidation states.
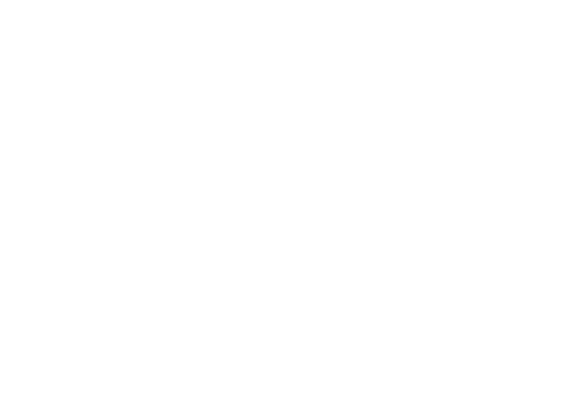
SUMMARY
Figure 3.21 Covalent adduct formation by flavin cofactors in enzymes. (A) Oxidation of dithiols to disulfides through formation of a C4a—dithiol complex, as in the reaction of glutathione reductase with dithiols. (B) Reoxidation of reduced flavin by molecular oxygen, with formation of a C4a—peroxide intermediate.
73
74 |
STRUCTURAL COMPONENTS OF ENZYMES |
|
|
Table 3.4 Some examples of cofactors found in enzymes |
|||
|
|
|
|
Cofactor |
Enzymatic Use |
Examples of Enzyme |
|
|
|
|
|
Copper ion |
Redox center—ligand binding |
Cytochrome oxidase, superoxide |
|
Magnesium ion |
Active site electrophile— |
dismutase phosphodiesterases, |
|
|
|
phosphate binding |
ATP synthases |
Zinc ion |
Active site electrophile |
Matrix metalloproteases, |
|
|
|
|
carboxypeptidase A |
Flavins |
|
Redox center—proton transfer |
Glucose oxidase, succinate dehydrogenase |
Hemes |
|
Redox center—ligand binding |
Cytochrome oxidase, cytochrome P450s |
NAD and NADP |
Redox center—proton transfer |
Alcohol dehydrogenase, ornithine cyclase |
|
Pyridoxal |
Amino group transfer— |
Aspartate transaminase, arginine |
|
phosphate |
stabilizer of intermediate |
racemase |
|
|
|
carbanions |
|
Quinones |
Redox center—hydrogen |
Cytochrome b , dihydroorotate |
|
|
|
transfer |
dehydrogenase |
Coenzyme A |
Acyl group transfer |
Pyruvate dehydrogenase, |
|
|
|
|
|
structural details of the enzyme active site facilitate substrate binding and the acceleration of reaction rates, which are the hallmarks of enzymatic catalysis.
REFERENCES AND FURTHER READING
Branden, C., and Tooze, J. (1991) Introduction to Protein Structure, Garland, New York.
Chotia, C. (1975) J. Mol. Biol. 105, 1.
Copeland, R. A. (1994) Methods for Protein Analysis: A Practical Guide to L aboratory Protocols, Chapman & Hall, New York.
Creighton, T. E. (1984) Proteins, Structure and Molecular Properties, Freeman, New
York.
Davis, J. P., and Copeland, R. A. (1996) Protein engineering, in Kirk-Othmer Encyclopedia of Chemical Technology, Vol. 20, 4th ed., Wiley, New York.
Dayoff, M. O. (1978) Atlas of Protein Sequence and Structure, Vol. 5, Suppl. 3, National Biomedical Res. Foundation, Washington, D.C.
Dill, K. A., Bromberg, S., Yue, K., Fiebig, K. M., Yee, D. P., Thomas, P. D., and Chan, H. S. (1995) Protein Sci., 4, 561.
Dixon, M., and Webb, E. C. (1979) Enzymes, 3rd ed., Academic Press, New York.
Dugas, H., and Penny, C. (1981) Bioorganic Chemistry, A Chemical Approach to Enzyme Action, Springer-Verlag, New York.
Hansch, C., and Coats, E. (1970) J. Pharm. Sci. 59, 731.
Klapper, M. H. (1977) Biochem. Biophys. Res. Commun. 78, 1018.
REFERENCES AND FURTHER READING |
75 |
Kyte, J., and Doolittle, R. F. (1982) J. Mol. Biol. 157, 105.
Pauling, L., Itano, H. A., Singer, S. J., and Wells, I. C. (1949) Science, 110, 543. Picot, D., Loll, P., and Garavito, R. M. (1994) Nature, 367, 243.
Stryer, L. (1989) Molecular Design of L ife, Freeman, New York. Walsh, C. (1979) Enzyme Reaction Mechanisms, Freeman, New York.
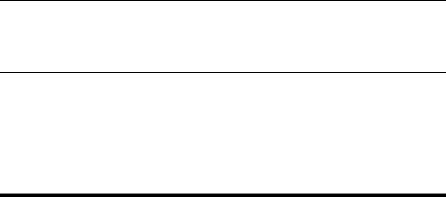
Enzymes: A Practical Introduction to Structure, Mechanism, and Data Analysis.
Robert A. Copeland Copyright 2000 by Wiley-VCH, Inc.
ISBNs: 0-471-35929-7 (Hardback); 0-471-22063-9 (Electronic)
4
PROTEIN--LIGAND
BINDING EQUILIBRIA
Enzymes catalyze the chemical transformation of one molecule, the substrate, into a different molecular form, the product. For this chemistry to proceed, however, the enzyme and substrate must first encounter one another and form a binary complex through the binding of the substrate to a specific site on the enzyme molecule, the active site. In this chapter we explore the binding interactions that occur between macromolecules, such as proteins (e.g., enzymes) and other molecules, generally of lower molecular weight. These binding events are the initiators of most of the biochemical reactions observed both in vitro and in vivo. Examples of these interactions include agonist and antagonist binding to receptors; protein—protein and protein—nucleic acid complexation; substrate, activator, and inhibitor binding to enzymes; and metal ion and cofactor binding to proteins. We shall broadly define the smaller molecular weight partner in the binding interaction as the ligand (L) and the macromolecular binding partner as the receptor (R). Mathematical expressions will be derived to describe these interactions quantitatively. Graphical methods for representing experimental data will be presented that allow one to determine the equilibrium constant associated with complex dissociation. The chapter concludes with a brief survey of experimental methods for studying protein—ligand interactions.
4.1 THE EQUILIBRIUM DISSOCIATION CONSTANT, Kd
We begin this chapter by defining the equilibrium between free (i.e., unoccupied) and ligand-bound receptor molecules. We will assume, for now, that the receptor has a single binding site for the ligand, so that any molecule of receptor is either free or ligand bound. Likewise, any ligand molecule must be
76
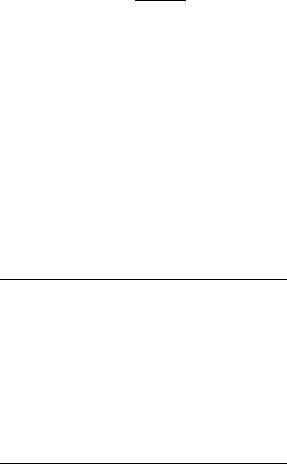
THE EQUILIBRIUM DISSOCIATION CONSTANT, Kd |
77 |
either free or bound to a receptor molecule. This assumption leads to the following pair of mass conservation equations:
[R] [RL] [R] |
(4.1) |
[L] [RL] [L] |
(4.2) |
where [R] and [L] are the total concentrations of receptor and ligand, respectively, [R] and [L] are the free concentrations of the two molecules, and [RL] is the concentration of the binary receptor—ligand complex.
Under any specific set of solution conditions, an equilibrium will be established between the free and bound forms of the receptor. The position of this equilibrium is most commonly quantified in terms of the dissociation constant, K , for the binary complex at equilibrium:
K |
[R] [L] |
(4.3) |
[RL] |
The relative affinities (i.e., strength of binding) of different receptor—ligand complexes are inversely proportional to their K values; the tighter the ligand binds, the lower the value of the dissociation constant. Dissociation constants are thus used to compare affinities of different ligands for a particular receptor, and likewise to compare the affinities of different receptors for a common ligand.
The dissociation constant can be related to the Gibbs free energy of binding for the receptor—ligand complex (Table 4.1) as follows:
G RT ln(K ) |
(4.4) |
The observant reader may have noted the sign change here, relative to the
Table 4.1 Relationship between Kd and Gbinding for receptor--ligand complexes at 25°C
K (M) |
G (kcal/mol) |
10 (mM) |
4.08 |
10 |
5.44 |
10 |
6.80 |
10 ( M) |
8.16 |
10 |
9.52 |
10 |
10.87 |
10 (nM) |
12.23 |
10 |
13.59 |
10 |
14.95 |
10 (pM) |
16.31 |
Calculated using Equation 4.4.
78 PROTEIN--LIGAND BINDING EQUILIBRIA
typical way of expressing the Gibbs free energy. This is because in general chemistry texts, the free energy is more usually presented in terms of the forward reaction, that is, in terms of the association constant:
G |
RT ln |
|
[RL] |
|
(4.5) |
|
[R] [L] |
||||||
|
|
|
||||
or |
|
|
|
|
|
|
G RT ln(K ) |
(4.6) |
The sign conversion between Equations 4.4 and 4.6 merely reflects the inverse relationship between equilibrium association and dissociation constants:
K |
1 |
(4.7) |
K
At this point the reader may wonder why biochemists choose to express affinity relationships in terms of dissociation constants rather than the more conventional association constants, as found in most chemistry and physics textbooks. The reason is that the dissociation constant has units of molarity and can thus be equated with a specific ligand concentration that leads to half-maximal saturation of the available receptor binding sites; this will become evident in Section 4.3, where we discuss equilibrium binding experiments.
4.2 THE KINETIC APPROACH TO EQUILIBRIUM
Let us begin by considering a simple case of bimolecular binding without any subsequent chemical steps. Suppose that the two molecules, R and L, bind reversibly to each other in solution to form a binary complex, RL. The equilibrium between the free components, R and L, and the binary complex, RL, will be governed by the rate of complex formation (i.e., association of the complex) and by the rate of dissociation of the formed complex. Here we will define the second-order rate constant for complex association as k and the first-order rate constant for complex dissociation as k .
R L RL |
(4.8) |
|
|
The equilibrium dissociation constant for the complex is thus given by the ratio of k to k :
K |
k |
|
(4.9) |
|
k |
||||
|
|
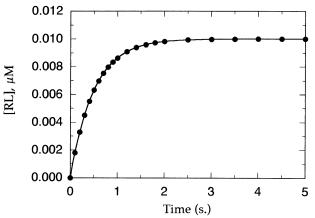
THE KINETIC APPROACH TO EQUILIBRIUM |
79 |
Figure 4.1 Time course for approach to equilibrium after mixing of a receptor and a ligand.
The data are fit by nonlinear regression to Equation 4.10, from which an estimate of the observed pseudo-first-order rate constant (k ) is obtained.
In the vast majority of cases, the strength of interaction (i.e., affinity) between the receptor and ligand is such that a large excess of ligand concentration is required to effect significant binding to the receptor. Hence, under most experimental conditions association to form the binary complex proceeds with little change in the concentration of free (i.e., unbound) ligand; thus the association reaction proceeds with pseudo-first-order kinetics:
[RL] [RL] [1 exp( k t) |
(4.10) |
where [RL] is the concentration of binary complex at time t, [RL] is the concentration of binary complex at equilibrium, and k is the experimentally determined value of the pseudo-first-order rate constant for approach to equilibrium. Figure 4.1 illustrates a typical kinetic progress curve for a receptor—ligand pair approaching equilibrium binding. The line drawn through the data in this figure is a nonlinear least-squares best fit to Equation 4.10, from which the researcher can obtain an estimate of k .
For reversible binding, it can be shown that the value of k is directly proportional to the concentration of ligand present as follows:
k k k [L] |
(4.11) |
Hence, one can determine the value of k at a series of ligand concentrations from experiments such as that illustrated in Figure 4.1. A replot of k as a function of ligand concentration should then yield a linear fit with slope equal to k and y intercept equal to k (Figure 4.2). From these values, the equilibrium dissociation constant can be determined by means of Equation 4.9.