
- •Copyright © 2006 by Oxford University Press, Inc.
- •Contents
- •1 Introduction
- •References
- •2.1 Macroscopic, Deterministic Chemical Kinetics
- •2.2 Disordered Kinetics
- •2.3 Fluctuations
- •References
- •3 A Brief Review of Methodology for the Analysis of Biochemical Reactions and Cells
- •3.1 Introduction
- •3.2 Measurement of Metabolite Concentrations
- •3.3 Principles and Applications of Mass Spectrometry
- •3.5 Fluorescent Imaging
- •3.6 Conclusions
- •References
- •4.1 Chemical Neurons and Logic Gates
- •4.2 Implementation of Computers by Macroscopic Chemical Kinetics
- •4.3 Computational Functions in Biochemical Reaction Systems
- •References
- •5.1 Theory
- •5.2 An Example: The Glycolytic Pathway
- •References
- •6 Experimental Test of the Pulse Perturbation Method for Determining Causal Connectivities of Chemical Species in a Reaction Network
- •Reference
- •Discussion
- •References
- •References
- •9 Density Estimation
- •9.1 Entropy Metric Construction (EMC)
- •9.2 Entropy Reduction Method (ERM)
- •References
- •10 Applications of Genetic Algorithms to the Determination of Reaction Mechanisms
- •10.1 A Short Primer on Genetic Algorithms
- •10.2 Selection of Regulation of Flux in a Metabolic Model
- •10.3 Evolutionary Development of Biochemical Oscillatory Reaction Mechanisms
- •10.5 Summary
- •References
- •11 Oscillatory Reactions
- •11.1 Introduction
- •11.2 Concepts and Theoretical Constructs
- •11.3 Experiments Leading to Information about the Oscillatory Reaction Mechanism
- •11.4 Examples of Deduction of Reaction Mechanism from Experiments
- •11.5 Limits of Stoichiometric Network Analysis
- •References
- •12.1 Lifetime Distributions of Chemical Species
- •12.2 Response Experiments and Lifetime Distributions
- •12.3 Transit Time Distributions in Complex Chemical Systems
- •12.4 Transit Time Distributions, Linear Response, and Extracting Kinetic Information from Experimental Data
- •12.5 Errors in Response Experiments
- •12.7 Conclusions
- •References
- •13.1 Clustering
- •13.2 Linearization in Various Forms
- •13.3 Modeling of Reaction Mechanisms
- •13.4 Boolean Networks
- •13.5 Correlation Metric Construction for Genetic Networks
- •13.6 Bayesian Networks
- •13.7 Some Other Illustrative Approaches
- •References
- •Index

1
Introduction
Chemical kinetics as a science has existed for more than a century. It deals with the rates of reactions and the details of how a given reaction proceeds from reactants to products. In a chemical system with many chemical species, there are several questions to be asked: What species react with what other species? In what temporal order? With what catalysts? And with what results? The answers constitute the macroscopic reaction mechanism. The process can be described macroscopically by listing the reactants, intermediates, products, and all the elementary reactions and catalysts in the reaction system.
The present book is a treatise and text on the determination of complex reaction mechanisms in chemistry and in chemical reaction systems that occur in chemical engineering, biochemistry, biology, biotechnology, and genomics. A basic knowledge of chemical kinetics is assumed.
Several approaches are suggested for the deduction of information on the causal chemical connectivity of the species, on the elementary reactions among the species, and on the sequence of the elementary reactions that constitute the reaction pathway and the reaction mechanism. Chemical reactions occur by the collisions of molecules, and such an event is called an elementary reaction for specified reactant and product molecules. A balanced stoichiometric equation for an elementary reaction yields the number of each type of molecule according to conservation of atoms, mass, and charge. Figure 1.1 shows a relatively simple reaction mechanism for the decomposition of ozone by light, postulated to occur in a series of three elementary steps. (The details of collisions of molecules and bond rearrangements are not discussed.) All approaches are based on the measurements of the concentrations of chemical species in the whole reaction system, not on parts, as has been the practice.
1
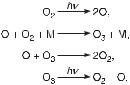
2DETERMINATION OF COMPLEX REACTION MECHANISMS
Fig. 1.1 Hypothesis for the elementary steps in the formation and decomposition reactions of ozone irradiated by light in the upper atmosphere.
One approach is called the pulse method, in which a pulse of concentration of one or more species of arbitrary strength is applied to a reacting system and the responses of as many species as possible are measured. From these responses causal chemical connectivities may be inferred. The basic theory is explained, demonstrated on a model mechanism, and tested in an experiment on a part of glycolysis.
In a second approach, measurements on time series of concentrations are used to construct correlation functions of concentrations. A theory is developed that shows that from these correlation functions information may be inferred on the reaction pathway, the reaction mechanism, and the centers of control in that mechanism. This method has been tested in experiments on glycolysis and has been applied to an extensive experimental genome study.
A third approach is based on the application of genetic algorithm methods to the study of the evolutionary development of a reaction mechanism, to the attainment of given goals (flux control, for example) in a mechanism, and to the determination of a reaction mechanism and rate coefficients by comparison with experiments.
Oscillatory reactions require a separate analysis, which is presented in detail. Responses of nonlinear systems to pulses or other perturbations are treated in some generality. The concluding chapter gives a brief introduction to bioinformatics, including several methods for determining reaction mechanisms.
There is not much literature (publications, texts, or treatises) on discussion of the methods and the art of guessing reaction mechanisms. In [1] there is a listing of hints and several rules that are helpful for developing a reaction mechanism for relatively simple systems. Once you have guessed a series of elementary steps, then there are two approximate methods that have been used extensively. One method is applicable for systems with a number of elementary reactions, one of which is slower than the others and hence is said to be rate-determining. The other is applicable for systems in which all the elementary reactions have about the same rate, but the time variation of intermediates is close to zero, the so-called stationary state hypothesis [1]. These two approximations have been very useful.
For more complex systems the approach used for many years, in its simplest description, has been the following: Identify as many species in the system as possible; separate the system into individual elementary steps; measure the stoichiometric coefficient for each species in each elementary step; determine the kinetics (the rate) of each elementary step; and postulate how the elementary steps may follow each other in sequence to produce the final products from the initial reactants. From this postulated reaction mechanism, you may derive kinetic equations and check these against available experiments. If there is agreement, then the mechanism is sufficient—it is a possible mechanism. In addition to this simple outline many other techniques have been used with great success: isotope measurements, mass spectrometry, and ion cyclotron methods, among many others (see chapter 3).
INTRODUCTION 3
The approaches and methods discussed here differ from the prior art described in the last paragraph. The attempt is to keep as much of the system together as possible in order to maintain as many of the connections as possible: the connections of the species to each other, the connections of the effectors on enzymes, and so on. The isolation of a single elementary step cuts many interactions, as seen in fig. 1.2: isolate the reaction fructose 6-phosphate to fructose 1,6-bisphosphate, and the interactions with fructose 2,6- bisphosphate, among many others, are severed. “To keep as much of the system together as possible” means to measure as many species as possible. The enormous progress in the instrumentation for the measurements of chemical species and their concentrations in the last twenty-five years now permits the simultaneous measurements of dozens to hundreds of species (see chapter 3); we need the construction of experiments and theories designed to deduce or infer, not guess, from such measurements information on the connectivity of the species, on the reaction pathway, and on the reaction mechanism.
As chemistry penetrates biology, biotechnology, and genome studies, the reaction systems being considered become more and more complex, with many more species, many more catalysts, more complex connectivities, and positive and negative feedforward and feedback interactions. New experiments and theories are necessary for investigating such systems, and this book is an introduction to the field. Much, but certainly not all, of the contents of the book is the result of our research studies in the last fifteen years, and it is time to assemble this work into a coherent presentation.
1.1Some Basic Definitions
We need to begin with several definitions of terms used throughout. (For an introduction to chemical kinetics see [1].)
Elementary reaction. The term “elementary reaction” has been defined. Sometimes two or more elementary reactions are added, as for example the elementary steps in a Michaelis–Menten enzymatic reaction, and listed as a pseudo-elementary reaction.
Catalyst. A catalyst may be necessary for an elementary reaction to occur at a reasonable rate, as well as positive and negative effectors on the catalyst. We shall be concerned mostly with isothermal reactions.
Reaction mechanism. A series of elementary reaction steps, usually postulated, that leads from stated reactants to products, including catalysts and effectors, is called the reaction mechanism for that overall reaction. An example of an important biological reaction mechanism, a part of glycolysis, is shown in fig. 1.2.
Reaction pathway. A listing of sequential chemical species in a reaction mechanism is called a reaction pathway; for example, the sequence glucose 6-phosphate, fructose 6-phosphate, fructose 1,6-bisphophate (see fig. 1.2) is a reaction pathway in the glycolysis reaction mechanism.
Causal connectivity. The direct connection of one species, say glucose, to another, glucose 6-phosphate, by reaction is called causal connectivity. A sequence of causal connectivities gives a reaction pathway from which a reaction mechanism, or parts thereof, may be inferred. There will be more on this subject later.
Control. A mechanism may be designed for control (regulation) of various functions: control of concentrations (homeostasis), of rates, of specific product formation, of temperature, and so on. We shall see some examples later.
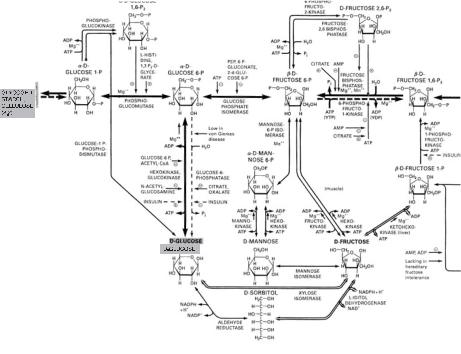
4DETERMINATION OF COMPLEX REACTION MECHANISMS
Fig. 1.2 Details of the reaction mechanisms of a part of glycolysis and the pentose cycle. Note the presence of the metabolites, enzymes, and the positive and negative effectors on the enzymes. Species will be defined in the later figures and in the text as needed. (Reproduced with permission from [8].)
Let us deal with the issue of uniqueness of a reaction mechanism. If a scientific theory, model, or hypothesis agrees with available experiments, then the agreement provides sufficiency, never necessity, for these theoretical constructs. In the literature of chemical kinetics there are ubiquitous warnings that the agreement of the predictions of a reaction mechanism with experiment does not prove that the mechanism is unique, that is, necessary, but only that it is a possible mechanism, it is sufficient. You can show that a mechanism is wrong by comparison with experiments; you cannot prove that it is necessary. These statements hold also for our approaches, as for all of science; they yield sufficient, not necessary, pathways and mechanisms.
1.2Introduction to Classical Identification
The purpose of this section is to expose the reader to the acknowledged scholarly field of deducing, or inferring, structure and dynamics from measurements, also called classical identification (see [2] and [3] for representative references). This is well known to
INTRODUCTION 5
chemical engineers, but strangely the topic cannot be found in the chemical literature and texts. Let us consider an example. Suppose you feed a batch culture of some bacteria with suitable nutrients at a given inflow rate, and you measure a series of molecular (concentrations of species) and physiological (pH, temperature) functions in time; you may also measure gene expression (protein production) and the production of some drug or secondary metabolite. Say that you wish to optimize the rate of production of the drug by varying the inflow of nutrients and varying concentrations of intermediates, pH, temperature, and so on. We can express the variations of the concentrations of the genes, the metabolites, and the drug by an equation in which general model structure is asserted. An example is a simple nonlinear regression in which the observation of one variation at time t is dependent on other variables at time (t − τ ), and may be expressed as:
Si = β0 + β1, j Sj (t − τ ) + |
β2, j, k Sj (t − τ )Sk (t − τ ) + · · · (1.1) |
j |
j k |
where the regression methods solve for the parameters β.
This example is relatively unstructured and methods like LISREL (reference in the next paragraph) allow the assertion of more causal hypotheses into the regression. We may simply search for the values of the parameters β which maximize the production of the desired drug, say S3, without seeking causal connections. We may also guess terms in this equation from prior knowledge or eliminate terms by designing gene deletion experiments and thus introduce causal relations. Clearly, as more and more causal structure is entered, sooner or later one arrives at a full mechanistic model. In all cases, the problem reduces to one of parameter estimation for the coefficients of the equations. In chapters 5, 6, and 12 we describe approaches based on perturbing a system, linear or nonlinear, measuring responses, and inferring information on the reaction mechanisms of the system.
Analysis by linear regression is the fit of given measurements by a straight line. The programs LISREL and SEPATH (see [4]–[7]) provide linear regression with some causal input. The linear or linearized response of a system to a small perturbation depends on the location of the system in the space of its variables. To obtain a linear response in a possibly nonlinear system requires small perturbations, and hence to be useful the errors in the measurements must be small—smaller than the perturbations. The issues of importance here are:
1.Observability. Perturb one species and see what you can determine, that is, measure, about the other species.
2.Controllability. For a given system, can you perturb at a point A in the space of the variables and from there come to another point B?
3.Reachability. Is the attainment of point B from point A feasible?
Kalman filtering is used for nonlinear systems either to control a given output or to fit a set of measurements by a linear fit over a small region of the measurements and vary that fit from one region to the next.
The classical identification becomes difficult or inapplicable when we have systems with a high degree of nonlinearity, when only partial observations can be made, and when there are stochastic (random) elements, that is, the variables are given by probability distributions rather than by given numbers. Multivariate systems in which only relatively inaccurate and imprecise measurements can be made, usually with insufficient time and