
- •Introduction
- •Materials and methods
- •Animals
- •Anatomical model
- •Histology
- •Measurements of pupil dynamics
- •Focal length measurements in the lower lens-eye
- •Method 1: point source imaging by isolated lenses
- •Optical investigation of the upper lens-eye
- •Results
- •Lower lens-eye morphology
- •Optics of the lower lens-eye
- •Upper lens-eye morphology
- •Optics of the upper lens-eye
- •Pit and slit eyes
- •Discussion
- •References
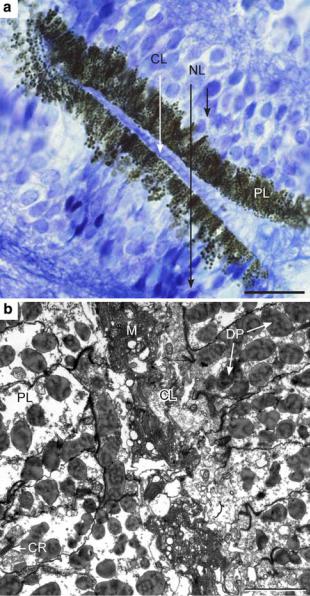
J Comp Physiol A (2009) 195:557–569 |
565 |
|
|
to light sources from the anterior or lateral sides of the optical axis. Illumination with parallel light from the posterior side of the optical axis did not produce a sharp shadow, partly because of a diffuse pigment edge at that side of the aperture, and partly because light from that direction passes through the balloon cells that scatters light (Fig. 7e) and dissolves the shadow. Another scattering structure in the upper lens-eyes is the cataract-like lens core, and with a point source, this was consistently seen to produce a very faint shadow on the retina (Fig. 7f).
Pit and slit eyes
The two other eye types, the pit and slit eyes, are much smaller than the lens eyes and do not contain lenses. The approximate dimension of the slit eye aperture is 80 9 20 lm and the circular aperture of the pit eye has a diameter of 30 lm. Like the situation in the lens eyes, the photoreceptors in the pit and slit eyes are pigmented and ciliary, and organised, as in the lens eyes, to form a ciliary layer, a pigment layer and a neural layer (Fig. 8a). The microvilli is partly running in wave-like bundles and partly more randomly entangled (Fig. 8b). The slit eye aperture does not contain the weakly scattering cells found in the slit eye of T. cystophora (Garm et al. 2008), but the aperture is instead filled with clear tissue, which likely serves a protective function (Fig. 8a). The pit eye does not contain similar tissue at its aperture, but there is instead an epithelium/cornea covering the eye.
Discussion
Box jellyfish have a surprisingly elaborate and intriguing visual system. All box jellyfish, including Chiropsella bronzie, have the same general organisation of their visual system, with the same number of eyes arranged in a stereotyped way on the rhopalia. Within this conserved arrangement, we here show that variations do exist between species on a more detailed level. Some variation between box jellyfish species is indeed to be expected because different species are found in different habitats, including sandy beaches, mangroves, kelp forests, open seas, intertidal regions and coral reefs (Coates 2003; Morandini 2003).
Unfortunately, the ecology of C. bronzie is not well studied. They are regularly found close to the shoreline, in knee-depth water along sandy beaches of North Queensland, Australia (Gordon et al. 2004), but they are frequently absent from these beaches throughout the season, suggesting that they move to different habitats. Additionally, the habitat of the polyps is unknown (Gordon et al. 2004) and the continual shifting of sand close to the shoreline of
Fig. 8 The slit eye of Chiropsella bronzie. a A light micrograph showing the general outline with the same layers as in the lens eyes: a ciliary layer (CL), a pigment layer (PL) with dark screening pigment, and a neural layer (NL) with receptor-cell nuclei. Scale bar 20 lm. b Transmission electron micrograph showing dark pigment granules (DP), numerous cross-sectioned cilia within and close to the microvillar mass (M), and a longitudinally cut rootlet (CR) in the pigment layer. Scale bar 2 lm
beaches is not a suitable place for polyps. Hence, it is most probable that C. bronzie has additional habitats, other than sandy beaches. These other habitats may include mangroves, which are prevalent in northern Queensland and/or deeper waters away from the beach.
More is known about the ecology of T. cystophora, which is also the only species in which the structure and function of the eyes are known well enough to allow a
123
566 |
J Comp Physiol A (2009) 195:557–569 |
|
|
comparison with the present results on C. bronzie. T. cystophora is a Carribean species which, during the day, is reliably found at the edge of mangrove lagoons, underneath the canopy. They use their eyes to avoid collision with mangrove prop roots (Garm et al. 2007) and they are visually attracted to light shafts penetrating the mangrove canopy (Buskey 2003). Although C. bronzie also performs visually-guided obstacle avoidance (Garm et al. 2007), the tasks for which C. bronzie and T. cystophora use their eyes may be at least partially different, and any differences in their eyes could potentially inform us about the roles of vision in the two species.
A retina covering at least the rear half of the lens in the lower lens-eyes of C. bronzie and T. cystophora, suggests that these eyes have very large visual fields (probably approaching 180L) in both species. But there are also species differences in the lower lens-eye. The lens is spherical in T. cystophora, but flattened in C. bronzie. The focal length in C. bronzie was found to be approximately 4.5 lens radii (radius taken perpendicular to the optical axis) which is considerably longer than the focal length of 2.6–3.7 lens radii in T. cystophora (Nilsson et al. 2005). In both species, the focal length is much too long for the retina to be in the focal plane, resulting in a severe underfocusing and blurred vision. This blurring is substantial in
T. cystophora, and even worse in C. bronzie.
The degree of image blurring is determined not only by the amount of under-focusing, but also by the pupil diameter, which changes in response to light in both species. In T. cystophora, the blur circle in the central visual field subtends an angle in space of 20 and 25L for constricted and dilated pupils, respectively (taken from Fig. 3c in Nilsson et al. 2005). In C. bronzie, the corresponding estimate is 20 and 52L (Fig. 5a), for the pupil diameter range of 77–210 lm. With aberration-free optics, the diameter of the out-of-focus blur circle would vary linearly with the pupil diameter, but because the lenses of both species suffer from positive spherical aberration, the shorter focal length of peripheral rays makes the blur circle grow less than the pupil diameter. Spherical aberration will thus act to keep the blur circle more constant, such that retinal light flux can be regulated without too much impact on the spatial resolution of the system.
Because the receptors are very narrow compared to the diameter of the blur circle, and the retinal depth is much shorter than the focal length of the lens, the angle that the blur circle will subtend at the nodal point (pupil centre) will correspond closely to the angular sensitivity of a single photoreceptor, and thus be a measure of the spatial resolution of the eye. Accordingly, the lower lens-eyes of both C. bronzie and T. cystophora will have a spatial resolution of at best 20L. This is an extremely poor spatial resolution, which is good enough only for detecting very large objects
at very close range. The simple modifications needed to put the retina in the focal plane of the lens, strongly suggest that the large blur circle is a deliberate adaptation to consistently remove fine spatial details from the image. Small camera-type eyes of other animals typically have a spatial resolution below 1L, and for larger eyes it can be much less than 1L (Land and Nilsson 2002). The optical design of the lower lens-eye of box jellyfish is thus not aimed at maximum acuity, but rather designed as an eye with a spatial low-pass filter tuned to detect only large structures at short range.
The lower lens-eye has been demonstrated to serve visual obstacle-avoidance in both species (Garm et al. 2007). However, there were differences in the performance of this behaviour between the two species. T. cystophora is a more agile swimmer and is better able to perform obstacle avoidance on smaller objects than is C. bronzie. The poorer obstacle avoidance ability of C. bronzie is likely explained by its more under-focussed optics found here. The sandy beaches, where C. bronzie are regularly found, contain much fewer obstacles than the edge of mangrove lagoons, where T. cystophora live. It is possible that the demands for an obstacle-avoidance response in these different habitats offer an explanation to the different optical performance. Interestingly, from descriptions and drawings of other box jellyfish eyes, it seems that T. cystophora and C. bronzie cover the range of lens shapes occurring in different cubozoan species. (Yamasu and Yoshida 1976; Martin 2004).
Even if there is a distinction in lens types in the lower lens-eye between different species of box jellyfish, it does not seem to have a major impact on the performance of the eyes. The retinal blur circle of the lower lens-eye of C. bronzie and T. cystophora are 20L or larger, and it is fair to ask if the lens is not at all needed for such a poor performance. From the geometry diagram in Fig. 5a, it is easy to calculate that if water was substituted for the lens, the retinal blur circle would grow only by 28% under an open pupil, and by even less, 14%, under a fully constricted pupil. Thus, the alarming conclusion is that the lens only has a marginal effect on spatial resolution, and with a constricted pupil, the lens has practically no optical function at all. As the pupil opens, resolution does not drop quite as fast as it would without the lens, and this can be interpreted as a way to allow sensitivity changes without the full corresponding change in resolution.
It seems a little far-fetched that a lens would initially evolve only to obtain these marginal effects on resolution. It is more likely that the original reason for the lens was to protect the retina and maintain the shape of the eye. The original function of the lens in the lower lens-eye of box jellyfish may thus be to act as a vitreous mass in a pigment cup eye. Once the cellular mass is there, it can of
123
J Comp Physiol A (2009) 195:557–569 |
567 |
|
|
course be engaged for fine-tuning of the eye’s sensitivity and spatial resolution, and from there, focusing may gradually take over as the main function. The optics of the lower lens-eye of C. bronzie, is closer to a pinhole eye than it is to a camera-type eye, and it thus represents an early stage of lens evolution, before focusing took over as the main role of the evolving lens. Because the evolution of vision is likely to begin with visual tasks that specifically require low resolution (Land and Nilsson 2006), and proceed to more advanced tasks requiring higher resolution, the lower lens-eye offers a unique insight into the early steps of lens evolution. However, it is not possible to tell if the difference between C. bronzie and T. cystophora indicate that one (C. bronzie) has retained more ancestral conditions, or if the differences are more recent results of tuning to the present habitats and visually guided behaviours.
The number of identifiable cell types in the retina of the lower lens-eye is a matter of some variation in the literature, probably depending on species differences. Some investigators found two cells types (Yamasu and Yoshida 1976), while others found three (Berger 1900). In this study of the C. bronzie, we identified two cell types making up the retina of the lower lens-eye; one pigmented sensory cell and one long pigment cell. Similar to the medusae of other Cubozoa (Yamasu and Yoshida 1976; Laska and Hu¨ndgen 1982; Martin 2004) as well as those of Hydrozoa (Eakin and Westfall 1962; Singla 1974; Singla and Weber 1982a, b) and Scyphozoa, the photoreceptor cells were identified, morphologically, as being ciliary. Recently, a number of cnidarian opsins have been sequenced (Plachetzki et al. 2007; Koyanagi et al. 2008; Kozmik et al. 2008; Suga et al. 2008). With an exception these opsins have been placed into a new cnidopsin family (Plachetzki et al. 2007), and the single opsin found in cubozoan eyes groups with the majority of other cnidarian opsins (Koyanagi et al. 2008; Kozmik et al. 2008).
The other cell type indentified in the lower lens-eye retina was the long pigment cell. This cell type is not known from T. cystophora, but it has been reported from the lower lens-eye in some other cubomedusae (Berger 1900; Yamasu and Yoshida 1976). However, the occurrence of white pigment in these cells has not been described before. Additionally, the process of pigment migration during light/dark adaptation has been suggested but not previously documented (Berger 1898; Martin 2002). We found that the dark pigment of the long pigment cells moves from the ciliary layer of the retina to the neural layer during dark adaptation, whereas the white pigment occupies both locations at all times. Observation of the dark-adapted retina through the dilated pupil of a live eye reveals the long pigment cells as tall white tubes with a dark centre (Fig. 3c). The retina is not easily observed
through the constricted pupil, but if a light-adapted eye is torn apart, pieces of the retina reveal a similar appearance as in dark-adapted eyes. The distal segments of the long pigment cells are thus reflecting white light also when they are filled with dark pigment. This is explained by the white pigment forming an external tube in the distal segment, and that the dark pigment migrates largely within this tube.
The mysterious white pigment pegs (distal segments) scatter light in the retina, and as such, they would have a negative effect on the retinal image in any well-focused eye. But in the lower lens-eye of C. bronzie, the array of white pigment pegs will only have a minor effect on resolution, because the under-focusing is so severe that some extra scattering will not make the blurry image much worse. White pegs crossing the retina, will of course also act as a tapetum lucidum to some degree, and make for a slightly higher sensitivity. But such a function would be much more plausible if the white pigment formed a layer behind the ciliary layer, rather than projecting as long pegs all the way to the distal retinal surface. Another more exotic possibility is that the long pigment cells constitute a second class of photoreceptors, picking up light through their distal tip (the dark centre in Fig. 3c), and that the migration of the dark pigment inside the cell regulates the sensitivity of the cell. However, our electron microscopic study did not reveal any potential receptive structures inside the cell that would support such an interpretation. The functions of the long pigment cells will remain elusive until expression of opsin and transduction proteins can be confirmed or ruled out.
The upper lens-eyes differ more fundamentally between
T. cystophora and C. bronzie. In T. cystophora, the lens is spherical and almost aberration-free (Nilsson et al. 2005), whereas in C. bronzie, the lens is ellipsoid in shape, very soft, and does not appear to have much refractive power. The upper lens-eye of T. cystophora is under-focused, and the spatial resolution is similar to the lower lens-eye of the same species. But in C. bronzie the upper lens-eye is quite incapable of forming images of extended objects. The shallow and oval pigment cup, together with the wide but optically ineffective lens, suggest that this eye is not just a spatial low-pass filter. Even though it does not form images of extended objects, it forms sharp shadow borders from point sources located in the anterior or lateral parts of its visual field (Fig. 7a–d). In the natural environment, the only light sources that would produce such shadows are the sun and the moon. The fact that the upper lens-eye is normally pointing upwards strengthens the hypothesis that it is involved in detecting the solar or lunar position. Hence, behavioural experiments are needed to explore the interesting possibility that C.bronzie may perform heliotaxis and/or lunartaxis to determine the direction to or away from the beach, or to keep a straight course while swimming.
123
568 |
J Comp Physiol A (2009) 195:557–569 |
|
|
On the posterior side, the upper lens-eye has an incomplete pigment screen, which prevents the eye from forming a sharp shadow of point sources in the posterior part of the visual field. In addition, light from this part of the visual field has to pass through the mass of scattering balloon cells, which further prevents a sharp shadow. It thus seems that the upper lens-eyes in C.bronzie have evolved to determine the position of point sources only in one direction from zenith. This could be interpreted as a way to determine the elevation of a point source, irrespective of its azimuth angle. However, it should be realised that a point source would only be detected close to the water surface, due to the poor visibility in near-shore waters.
The cataract-like inclusions observed in the lens core of the upper lens-eye causes a small, diffuse, shadow on the retina when illuminated by a point source. Compared to the sharp, high contrast shadow that the eye’s pigment aperture cast from point sources, the shadow produced by the lens inclusion is of very low contrast. Therefore, although possible, it seems unlikely that the inclusion or its shadow has any functional significance.
The paired pit and slit eyes make up the lesser eyes of the visual system of box jellyfish. They are both considerably smaller than the lens-eyes and lack any lens-like structure. The function of the lesser eyes remains elusive, but considering their size and structure, it is likely that they just monitor the ambient intensity in specific regions of the visual environment and consequently, may play a role in particular phototactic behaviour. Interestingly, there are differences in the structure of the slit eye between C. bronzie and T. cystophora (Garm et al. 2008). The slit eye in T. cystophora contains vitreous cells that scatter light entering the eye, and the upper pigment edge extends much further than the lower edge. In C. bronzie, there are no scattering cells, and the upper and lower pigment edges extend about equally far out (Garm et al. 2008). It is not clear if these structural differences also imply functional differences between the species.
This investigation has demonstrated that even if different species of box jellyfish have the same number of eyes, there are differences to the eyes that indicate both quantitative and qualitative differences in the way they control the behaviour. The upper lens-eye is an excellent example where the basic design has been modified to allow image vision in one species and monitoring of point source elevation in another species. Even if the visual tasks supported by the eyes amongst box jellyfish species may differ to hold that each eye is specialised to support only one or a few visual tasks, and that complex visual behaviour results from the combined action of all four eye types.
Acknowledgments The authors would like to thank Jamie Seymour, Matthew Gordon and Teresa Carrette at James Cook
University, Cairns for their help and support in the field and for fruitful discussions. A. G. would like to acknowledge grant # 2005-1- 74 from the Carlsberg Foundation and D.-E.N. would like acknowledge grants from the Swedish Research Council and the Swedish Foundation for Strategic Research (BioX). All of the experiments conducted in this study comply with the ‘Principles of animal care’, publication no. 86-23, revised 1985 of the National Institute of Health and with the current laws for animal care in Australia and Sweden.
References
Berger EW (1898) The histological structure of the eyes of cubomedusae. J Comp Neurol 8:223–230
Berger EW (1900) Physiology and histology of the cubomedusae including Dr. F. S. Conant’s notes on the physiology. Mem Biol Lab Johns Hopkins Univ IV(4):1–84
Buskey EJ (2003) Behavioral adaptations of the cubozoan medusa
Tripedalia cystophora for feeding on copepod (Dioithona oculata) swarms. Mar Biol 142:225–232
Claus C (1878) Ueber Charybdea marsupialis. Arb aus der Zool Inst der Univ Wien II:16–55
Coates M (2003) Visual ecology and functional morphology of cubozoa (Cnidaria). Intergr Comp Biol 43:542–548
Coates MM, Garm A, Theobald JC, Thompson SH, Nilsson D-E (2006) The spectral sensitivity of the lens eyes of a box jellyfish, Tripedalia cystophora (Conant). J Exp Biol 209:3758–3765
Conant FS (1898) The cubomedusae. Mem Biol Lab Johns Hopkins Univ IV(1):1–61
Eakin RM, Westfall JA (1962) Fine structure of photoreceptors in hydromedusan, Polyorchis penicillatus. Proc Natl Acad Sci USA 48:826–833
Garm A, O’Connor M, Parkefelt L, Nilsson D-E (2007) Visually guided obstacle avoidance in the box jellyfish Tripedalia cystophora and Chiropsella bronzie. J Exp Biol 210:3616–3623 Garm A, Andersson F, Nilsson D-E (2008) Unique structure and optics of the lesser eyes of the box jellyfish Tripedalia
cystophora. Vision Res 48:1061–1073
Gordon M, Hatcher C, Seymour J (2004) Growth and age determination of the tropical Australian cubozoan Chiropsalmus sp. Hydrobiologia 530(531):339–345
Koyanagi M, Takano K, Tsukamoto H, Ohtsu K, Tokunaga F, Terakita A (2008) Jellyfish vision starts with cAMP signaling mediated by opsin-Gs cascade. Proc Natl Acad Sci 105(40): 15576–15580
Kozmik Z, Ruzickova J, Jonasova K, Matsumoto Y, Vopalensky P, Kozmikova I, Strnad H, Kawamura S, Piatigorsky J, Paces V, Vlcek C (2008) Assembly of the cnidarian camera-type eye from vertebrate-like components. Proc Natl Acad Sci USA 105: 8989–8993
Land MF, Nilsson D-E (2002) Animal eyes. Oxford University Press, New York
Land MF, Nilsson D-E (2006) General purpose and special purpose visual systems. In: Warrant EJ, Nilsson D-E (eds) Invertebrate vision. Cambridge University Press, Cambridge, pp 167–210
Laska G, Hu¨ndgen M (1982) Morphologie und ultrastruktur der lichtsinnesorgane von Tripedalia cystophora Conant (Cnidaria, Cubozoa). Zool Jb Anat 108:107–123
Martin VJ (2002) Photoreceptors of cnidarians. Can J Zool 80:1703– 1722
Martin VJ (2004) Photoreceptors of cubozoan jellyfish. Hydrobiologia 530(531):135–144
Morandini A (2003) Deep-sea medusae (Cnidiaria: Cubozoa, Hydrozoa and Scyphozoa) from the coast of Bahia (western South Atlantic, Brazil). Mitt Hamb Zool Mus Inst 100:13–25
123
J Comp Physiol A (2009) 195:557–569 |
569 |
|
|
Nilsson D-E, Gilse´n L, Coates M, Skogh C, Garm A (2005) Advanced optics in a jellyfish eye. Nature 435:201–205
Plachetzki DC, Degnan BM, Oakley TH (2007) The origins of novel protein interactions during animal opsin evolution. PLoS ONE. doi:10.1371/journal.pone.0001054
Schewiakoff W (1889) Beitra¨ge zur kenntnis des acalephenauges. Morpholog Jahrb XV:21–60
Singla CL (1974) Ocelli of hydromedusae. Cell Tissue Res 149: 413–429
Singla CL, Weber C (1982a) Fine-structure of the ocellus of Sarsia tubulosa (Hydrozoa, Anthomedusae). Zoomorphology 100:11–22
Singla CL, Weber C (1982b) Fine-structure studies of the ocelli of Polyorchis penicillatus (Hydrozoa, Anthomedusae) and their connection with the nerve ring. Zoomorphology 99:117–129
Skogh C, Garm A, Nilsson D-E, Ekstro¨m P (2006) Bilaterally symmetrical rhopalial nervous system of the box jellyfish Tripedalia cystophora. J Morphol 267:1391–1405
Suga H, Schmid V, Gehring WJ (2008) Evolution and functional diversity of jellyfish opsins. Curr Biol 18:51–55
Yamasu T, Yoshida M (1976) Fine-structure of complex ocelli of a cubomedusan, Tamoya bursaria Haeckel. Cell Tissue Res 170:325–339
123