
Yang Fluidization, Solids Handling, and Processing
.pdf
196 Fluidization, Solids Handling, and Processing
calculated separately, rather a pseudo-homogeneous convective coefficient hc is sought. Attention is thus focused on the “boundary layer” of the two-phase mixture at the heat transfer surface. Different approaches are used by various authors to represent the essence of the thermal transport process in this layer. The simplest form of this approach is to borrow from single phase convective correlations, and attempt to find general empirical relationships between an effective convective Nusselt number and some appropriate Reynolds number. Equations (17–21) represent examples of this approach, with their concurrent advantage of simplicity and disadvantage of limited applicability.
Martin (1984) suggested a more mechanistically based approach, utilizing analogy between kinetic motion of molecules in gases and particle motion in the FFB suspension. By drawing on the kinetic theory of gases, Martin developed a model to account for thermal energy transport across the boundary layer by particle motion. The resulting Nusselt number for particle-enhanced convection is obtained as
Eq. (25) |
N u c |
≡ |
hc d p = ε s (Z ) (1- e- Νwp /CZ ) |
|
|
|
|
kg |
|
where: |
εs |
= average solid volume fraction in bed |
||
|
Z |
= |
ρ p C pp d p |
∙ wp |
|
6 k g |
|||
|
|
|
|
|
|
Nwp |
= Nusselt number for wall-particle heat transfer |
||
|
wp |
= Average velocity of random particle motion |
The wall-particle Nwp represents the heat transfer process between the wall and a contacting particle. For gas-solid systems, where thermal resistance of the gas gap dominates,
|
~ 4 |
é(1+ |
|
)· |
æ1 + |
1 |
ö |
-1ù |
Eq. (26) N wp |
K n |
|
÷ |
|||||
|
ê |
|
ln ç |
|
ú |
|||
|
|
ë |
|
|
è |
K n ø |
û |
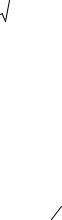
where:
Eq. (27)
where:
|
|
|
|
|
|
|
Heat Transfer in Fluidized Beds 197 |
|||
Kn = Knudsen number for gas in gap |
||||||||||
|
|
|
|
|
|
|
k g |
|
|
|
|
|
4 |
æ |
2 |
|
ö |
2πRT/ M g |
|||
|
= |
|
ç |
|
- 1 |
÷ |
|
|
|
|
Kn |
|
|
P (2C pg - R/ M g ) |
|||||||
|
|
ç |
γ |
|
÷ |
|||||
|
|
d p è |
|
ø |
γ = accommodation constant (» - 0.9 for air at ambient temperature)
Using Maxwell’s distribution for particle velocity, Martin obtained the following expression for the average random particle velocity as a function of particle size and concentration,
|
|
|
æ |
gd |
|
|
ε |
|
- ε |
1 |
||
|
|
|
p |
|
s ,mf |
ö 2 |
||||||
Eq. (28) |
w p |
= |
ç |
|
|
· |
|
|
s |
÷ |
||
5ε |
|
|
|
ε |
|
|
||||||
|
|
|
ç |
s ,mf |
|
|
s |
÷ |
||||
|
|
|
è |
|
|
|
|
ø |
The parameter C in Eq. (25) is a dimensionless parameter inversely proportional to the average residence time of single particles on the heat transfer surface. It is suggested that this parameter be treated as an empirical constant to be determined by comparison with actual data in fast fluidized beds. The lower two dash lines in Fig. 17 represent predictions by Martin’s model, with C taken as 2.0 and 2.6. It is seen that an appropriate adjustment of this constant would achieve reasonable agreement between prediction and data.
An alternate modeling approach is that suggested by Molerus (1993) and Wirth (1993, 1995). These researchers reasoned that the wall-to- suspension heat transfer in FFBs depends on the fluid mechanics immediately near the wall. They further conclude from experimental investigations that there is a thin layer near the wall of low solid concentration, providing significant thermal resistance between the wall and falling strands of relatively high solid concentrations. Consequently Wirth and Molerus sought to describe the Nusselt number for heat transfer between the wall and the two-phase suspension in terms of dimensionless numbers which characterize the gas-particle flow near the wall. They report that a dimensionless pressure-drop number which measures bed-averaged solid concentration, and the Archimedes number for the particle/gas system are sufficient to

198 Fluidization, Solids Handling, and Processing
correlate the convective heat transfer coefficient over wide ranges of fluid properties and operating conditions. At low Archimedes numbers, heat transfer occurs primarily by gas conduction. At higher Archimedes numbers, gaseous conduction and convection both contribute to heat transfer. The correlation suggested by Wirth (1995) simply assumes that conduction and convection are additive, resulting in the following correlation.
|
hc d p |
é |
DP/DH |
ù 0.5 |
|
|||
Eq. (29) |
= 2.85 ê |
ú |
+ 3.28 ´ 10 - 3 |
Recl Prg |
||||
|
|
|
||||||
|
k g |
ëê g (ρ s - ρ g )(ε s , mf )ûú |
|
|
||||
where: |
Recl |
= Reynolds number for falling cluster strands |
ρg d pU cl
=μ g
Ucl = velocity of falling cluster strands
Based on some physically reasoned assumptions, Wirth (1990) obtained the following relations for estimating Reynolds number of the falling strands:
Eq. (30) |
|
|
|
Re cl = |
|
|
2 (1 - φ ) 2 Re mf +φ Re t |
|
||||||||
|
|
|
|
|
2 [φ + (1 - φ )ε gmf ] |
|
|
|
||||||||
|
|
|
|
|
|
|
|
|
|
|
|
|||||
ìé |
(1 |
- φ )4 Re 2 |
+ (1 - φ )2 φRe |
|
|
|
ù |
(ε s ,mf |
½ |
|||||||
íê1 - 4 |
t |
Re |
mf |
ú -189 |
)φ 3 (1 - φ )Ar ] |
|||||||||||
îë |
|
|
|
mf |
|
|
|
|
|
|
û |
|
|
|
||
[2 (1 - φ )2 Re |
|
+ φRe |
|
-1 |
ü |
|
|
|
|
|
|
|
||||
mf |
t |
] |
+ 1 |
|
|
|
|
|
|
|
||||||
|
|
|
|
|
|
|
ý |
|
|
|
|
|
|
|
||
|
|
|
|
|
|
|
|
|
þ |
|
|
|
|
|
|
|

Heat Transfer in Fluidized Beds 199
where:
(1 - φ )= |
2.3 |
P/ |
H |
|
||
(ρ |
s |
- ρ |
) |
|
g |
|
|
|
|
g ε s,mf |
|
ρ g u g ,mf d p
Remf ≡
μg
=[1.14 ×103 + 0.04 Ar]1/2 - 33.7
Ret ≡ ρ g ut d p
μg
Figure 27 shows a comparison of Wirth’s correlation with experimental data for particles of various materials and diameter, in FFBs operating at various pressures. While the log-log plot submerges numerical differences, the agreement is generally good.
Models for estimating the radiative heat transfer contribution in fast fluidized beds parallel those proposed for dense bubbling beds, as reviewed above. The more mechanistically based approach of treating the particlegas medium as a radiative participative medium has been attempted by only a few researchers for FFBs. Chen, Cimini and Dou (1988) used the twoflux radiative model of Eqs. (12–13) to analyze simultaneous convection and radiation in high temperature FFBs. Turbulent convection was combined with decreet radiative fluxes in the three transport equations, including a term for volumetric heat generation. Sample results for fluidized combustor conditions predicted a significant interdependence of radiative and convective heat transfer. This conclusion was verified by the experimental measurements of Han (1992) who showed that the contribution of radiative heat transfer was strongly effected by bed-averaged solid concentration, decreasing with increasing solid mass flux and decreasing gas velocity.
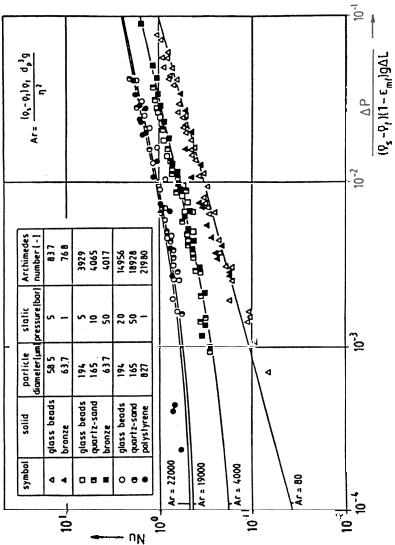
200 Fluidization, Solids Handling, and Processing
.
\ |
03 |
|
4~ |
-C" |
!~ |
-=: |
4 |
0.- 1 |
|
c>' |
|
I |
~ |
2 |
|
" |
|
.. |
|
c[ |
|
|
\ ~ |
,..m
'.;oD
~,..
|
|
|
|
|
|
|
4 |
|
~ |
|
|
I |
|
|
|
|
-a |
|
..~.. |
|
|
|
|
~ |
0 |
|
"O~c: |
|
|
|
|
.. |
|
~a.. I |
>~- |
|
|
|
|
a |
.D |
.. |
.DN- |
|
|
|
|
|
|
|
|
|
|||
... |
~ ... |
|
|
|
|
|
|
|
~ |
c |
|
|
|
|
|
|
o |
o |
|
|
. |
|
|
|
O'.D |
i~i |
|
|
|
||
"0 |
|
|
|
8 |
|
9 |
|
.0 |
|
|
|
8 |
|
||
e |
|
|
|
N |
o |
0 |
|
... |
|
|
|
N |
~ |
... |
«) |
... |
|
|
|
" |
" |
" |
" |
|
|
|
|
||||
|
|
|
|
.. |
.. |
.. |
.. |
|
|
|
|
|
c( |
c( |
0( |
|
|
~- |
|
o |
|
|
00 |
|
|
0 |
|
|
|
||
|
|
|
O |
|
|
||
|
|
- |
|
|
|
|
|
|
|
|
-nN |
|
|
|
|
-1
<:1
01
e
\.I
I
..-
0- I
..
~
..,
'0
"-
...
I
-~
-0
Figure 27. Comparison of model of Wirth with experimental data. (From Wirth, 1995.)
Heat Transfer in Fluidized Beds 201
The more common approach is to treat the particle-gas suspension as an equivalent gray surface parallel to the heat transfer surface. Equation (11) would than be used with F taken as unity. Grace (1986) suggests that the emissivity of the particle-gas suspension can be approximated as,
Eq. (31) eb ¾ 0.5(1 + es )
where es is the surface emissivity of the solid particles.
This gray body approach, or some modified version, has been the more popular approach used by various authors, including Palchonok et al., (1995), Anderson and Lechner (1993), Mahalingan and Kolar (1990).
Similar models using a multi-flux analysis of particle absorption and scattering are reported by Radauer, Glatzer and Linzer (1996).
NOTATIONS
A |
Volumetric absorption coefficient of radiation |
Ar |
Archimedes number, Eq. (1) |
CD |
Drag coefficient for single particle |
Cp |
Heat capacity |
d, D |
Diameter |
Dt |
Tube diameter |
e |
Radiative emissivity |
e |
Volumetric concentration (fraction) of a phase |
F |
View factor for radiation |
fl |
Time fraction of lean phase |
GMass flux per unit flow area g Gravitational acceleration
HHeight in bed
h effective heat transfer coefficient
IForward radiative flux, Eq. (12)
JBackward radiative flux, Eq. (12) k Thermal conductivity
LLength
MMolecular weight
μViscosity
Nu Nusselt number, Eq. (7)
202 Fluidization, Solids Handling, and Processing
P |
Pressure |
Pr |
Prandtl number |
R |
Radius of fluidized bed |
r |
radial distance from centerline |
S |
Volumetric scattering coefficient of radiation |
ρDensity
τResidence time
T Stefan Boltzmann constant of radiation u Superficial gas velocity
y Distance from wall surface
Subscripts
bBed
cConvective
cl |
cluster |
d |
Dense phase |
g |
Gas |
l |
Lean phase |
mf |
Minimum fluidization |
p |
Particle |
pa |
Particle packet |
rRadiative
sSolid
susp Solid-gas suspension t Terminal
w Wall
REFERENCES
Anderson, B. A., and Lechner, B., “Local Lateral Distribution of Heat Transfer on Tube Surface of Membrane Walls in CFB Boiler,” Circ. Fluid. Bed Tech IV, 311–318 (1993)
Baerg, A, Klasson, J., and Gishler, P. E., “Heat Transfer in a Fluidized Solids Bed,” Can. J. of Res., 28(8):287 (1950)
Baskakov, A. P., Berg, B. V., Vitt, O. K., Fillipovsky, N. F., Kirakosyn, V. A., Coldobin, J. M., and Maskaev, V. K., “Heat Transfer to Objects Immersed in Fluidized Bed,” Powder Tech., 8(5–6):273–282 (1973)
Heat Transfer in Fluidized Beds 203
Baskakov, A. P., “The Mechanism of Heat Transfer Between a Fluidized Bed and a Surface,” Int. Chem. Eng., 4:320 (1984)
Basu, P., and Nag, P. K., “Heat Transfer to Walls of a Circulating Fluidized Bed Furnace,” Chem. Eng. Sci., 1:1–26 (1996)
Beaude, F., and Louge, M., “Similarity of Radial Profiles of Solid Volume Fraction in a Circulating Fluidized Bed,” Fluidization VIII, pp. 245–253 (1995)
Berg, B. V., and Baskakov, A. P., “Investigation of Local Heat Transfer Between a Fixed Horizontal Cylinder and a Fluidized Bed,” International Chem. Eng., 14(3):440–443 (1974)
Bhattacharya, and Harrison, D., “Heat Transfer in High Temperature Fluidized Beds,” Proc. Euro. Conf. In Particle Tech., K7 (1976)
Biyikli, S., and Chen, J. C., “Effect of Mixed Particle Sizes on Local Heat Transfer Coefficients Around a Horizontal Tube in Fluidized Beds,” 7th Int. Heat Transfer Conf., Munich, Germany (1982)
Bock, H. J., and Schweinzer, J., “Heat Transfer to Horizontal Tube Banks in a Pressurized Gas\Solid Fluidized Bed,” German Chem. Eng., 9(1):16–23 (1986)
Borodulya, V. A., Teplitsky, Y. S., Sorokin, A. P., Matsnev, V. V., Markevich, I. I., and Kovenskiy, V. I., “External Heat Transfer in Polydispersed Fluidized Beds at Increased Temperatures,” J. Eng. Phys., 56(5):767–773 (1989)
Borodulya, V. A., Teplitsky, Y. S., Sorokin, A. P., Markevich, I. I., Hassan, A. F., and Yeryomenko, T. P., “Heat Transfer Between a Surface and a Fluidized Bed: Consideration of Pressure and Temperature Effects,” Int. J. of Heat and Mass Transfer, 34(4):47–53 (1991)
Botterill, J. S. M., Butt, M. H. D., Cain, G. L., and Redish, K. A., Inter. Symp. on Fluidization, p. 442, Univ. Press, Amsterdam, Netherlands (1967)
Botterill, J. S. M., and Williams, J. R., “The Mechanism of Heat Transfer to GasFluidized Beds,” Trans. Inst. Chem. Eng., 41:217–230 (1963)
Botterill, J. S. M., and Desai, M., “Limiting Factors in Gas-Fluidized Bed Heat Transfer,” Powder Technol., 6(4):231–238 (1972)
Brewster, M. O., and Tien, C. L., “Radiative Heat Transfer in Packed Fluidized Beds: Dependent Versus Independent Scattering,” J. Heat Transfer, 104(4):574–580 (1982)
Burki, V., Hirschberg, B., Tuzla, K., and Chen, J. C., “Thermal Development for Heat Transfer in Circulating Fluidized Beds,” ALChE Annual Meeting, (1993)
Chandran, R., Chen, J. C., and Staub, F. W., “Local Heat Transfer Coefficients Around Horizontal Tubes in Fluidized Beds,” J. of Heat Transfer, 102(2):152–157 (1980)
204 Fluidization, Solids Handling, and Processing
Chen, J. C., “Heat Transfer to Tube in Fluidized Beds,” Invited Lecture, Paper No. 76-HT-75, National Heat Transfer Conf., St. Louis, MO., (1976)
Chen, J. C., Chen, K. L., “Analysis of Simultaneous Radiative and Conductive Heat Transfer in Fluidized Beds,” Chem. Eng. Commun., 9:255–271 (1981)
Chen, J. C., Cimini, R. J., and Dou, S. H., “A Theoretical Model for Simultaneous Convective and Radiative Heat Transfer in Circulating Fluidized Beds,”
Circ. Fluid. Bed Tech. II, 255–262 (1988)
Chen, J. C., “Thomas Baron Plenary Lecture – Clusters,” AIChE Symp. Series, 92(313):1–6 (1969)
Chen, J. C., “Heat Transfer to Tubes in Fluidized Beds, “ Invited Lecture, Paper No. 76-HT-75, National Heat Transfer Conf., St. Louis (1976)
Cimini, R. J., and Chen, J. C., “Experimental Measurement of Radiant Transmission Through Packed and Fluidized Media,” Experimental Heat Transfer, I:45– 56 (1987)
Divilio, R. J., and Boyd, T. J., “Practical Implications of the Effect of Solids Suspension Density on Heat Transfer in Large-Scale CFB Boilers,” Circ. Fluid. Bed Tech IV, 334–339 (1993)
Dou, S., Herb, B., Tuzla, K., and Chen, J. C., “Heat Transfer Coefficients for Tubes Submerged in Circulating Fluidized Bed,” Experimental Heat Transfer, 4:343–353 (1991)
Dou, S., Herb, B., Tuzla, K., and Chen, J. C., “Dynamic Variation of Solid Concentration and Heat Transfer Coefficient at Wall of Circulating Fluidized Bed,” Fluidization VII, 793–801 (1993)
Dow, W. M., and Jacob, M., “Heat Transfer Between a Vertical Tube and A Fluidized Air-Solid Mixture,” Chem. Eng. Progr., 47(12):637–648 (1951)
Ebert, T., Glicksman, L., and Lints, M., “Determination of Particle and Gas Convective Heat Transfer Components in Circulating Fluidized Bed,” Chem. Eng. Sci., 48:2179–2188 (1993)
Einshtein, V. G., and Gelperin, N. I., “Heat Transfer Between a Fluidized Bed and a Surface,” Intern. Chem. Eng., 6(1):67–81 (1966)
Ergun, S., “Fluid Flow Through Packed Columns,” Chem Eng. Progress, 48(2):89– 94 (1952)
Fox, R. W., and McDonald, A. T., Introd. to Fluid Mechanics, 4:444, John Wiley & Sons, New York (1992)
Fraley, L. D., Lin, Y. Y., Hsiao, K. M., and Solbakken, A., ASME Paper 83-HT-92 (1983)
Furchi, J. C. L., Goldstein, L., Jr., Lombardi, G., and Mohseni, M., “Experimental Local Heat Transfer in a Circulating Fluidized Bed,” Circ. Fluid. Bed Tech. II, 263–270 (1988)