

Supplement A3: The Chemistry of Double-Bonded Functional Groups. Edited by Saul Patai Copyright 1997 John Wiley & Sons, Ltd.
ISBN: 0-471-95956-1
CHAPTER 5
Chiroptical properties of compounds containing C=O groups
STEFAN E. BOIADJIEV and DAVID A. LIGHTNER
Department of Chemistry, University of Nevada, Reno, Nevada 89557, USA Fax: 702-784-6804; e-mail: LIGHTNER@UNR.EDU
I. INTRODUCTION . . . |
. . |
. . . . . . . . . . . . . . . . . . . . . . . . . . . . . . . . |
155 |
II. THE OCTANT RULE |
!. . |
. Ł. .Transition. . . . . . .in. .Ketones. . . . . . . . . . . . . . . . . . . . |
156 |
D |
|
||
A. The Ketone C O n |
|
|
|
and Aldehydes . . . |
. . |
. . . . . . . . . . . . . . . . . . . . . . . . . . . . . . . . |
160 |
B. CDO Symmetry Planes, Quadrants and The Third Nodal Surface . . . . |
160 |
||
C. Front Octants and Anti-Octant Effects . . . . . . . . . . . . . . . . . . . . . |
161 |
||
D. Octant Consignate and Dissignate Perturbations . . . . . . . . . . . . . . . |
165 |
||
E. Qualitative Completeness . . . . . . . . . . . . . . . . . . . . . . . . . . . . . . |
166 |
||
F. Isotopic Perturbers |
. . |
. . . . . . . . . . . . . . . . . . . . . . . . . . . . . . . . |
168 |
G. Applications of the Octant Rule . . . . . . . . . . . . . . . . . . . . . . . . . . |
170 |
||
III. COMPILATION OF KETONE AND ALDEHYDE CD . . . . . . . . . . . . |
175 |
||
A. Acyclic Ketones and Aldehydes . . . . . . . . . . . . . . . . . . . . . . . . . |
175 |
||
B. Cyclic Ketones and Aldehydes . . . . . . . . . . . . . . . . . . . . . . . . . . |
178 |
||
IV. UNSATURATED KETONES . . . . . . . . . . . . . . . . . . . . . . . . . . . . . . |
202 |
||
A. ˛,ˇ-Unsaturation . |
. . |
. . . . . . . . . . . . . . . . . . . . . . . . . . . . . . . . |
202 |
B. ˇ, -Unsaturation and the Extended Octant Rule . . . . . . . . . . . . . . . |
203 |
||
V. COMPILATION OF UNSATURATED KETONE |
|
||
AND ALDEHYDE CD |
. |
. . . . . . . . . . . . . . . . . . . . . . . . . . . . . . . . |
206 |
VI. EXCITON CHIRALITY . |
. . . . . . . . . . . . . . . . . . . . . . . . . . . . . . . . |
240 |
|
VII. ADDENDUM . . . . . . |
. . |
. . . . . . . . . . . . . . . . . . . . . . . . . . . . . . . . |
250 |
VIII. ACKNOWLEDGMENTS |
. . . . . . . . . . . . . . . . . . . . . . . . . . . . . . . . |
252 |
|
IX. REFERENCES . . . . . |
. . |
. . . . . . . . . . . . . . . . . . . . . . . . . . . . . . . . |
252 |
|
|
|
|
I. INTRODUCTION
Chiroptical properties of compounds containing a carbonyl group are among the most studied in organic chemistry. Among the many different carbonyl-containing functional
155

156 |
Stefan E. Boiadjiev and David A. Lightner |
groups, in this series chiroptical properties of carboxylic acids and their simple derivatives (esters, lactones, amides, lactams) and thio analogues have been reviewed most recently in 19921. This review also included a section on exciton chirality. Chiroptical properties of ketones and aldehydes, on the other hand, have not been reviewed in this series, except for ˛,ˇ-unsaturated ketones2. Since Djerassi’s book on optical rotatory dispersion (ORD) appeared in 19603, many monographs have appeared covering chiroptical properties of organic chromophores4 15. Most of these appeared in the 1960s and 1970s, whereas from 198213 until 199414,15 none appeared. Since the mid-1960s the most studied chiroptical property of ketones and aldehydes has been circular dichroism (CD), and while books on CD have appeared periodically, they have covered many different aspects of CD spectroscopy.
Since the chiroptical properties of most compounds containing CDO groups have been reviewed recently in this series, in the following we focus on CD of ketones and the relatively few aldehydes that have been studied by CD spectroscopy. Because most studies of saturated ketones have focussed on their octant rule behavior, a description of the octant rule and how to apply it appears early in this chapter. This is followed by an easy-to- read graphic compilation of the published work on saturated aldehydes and ketones since 1977, the date of the most recent comprehensive review10 with many literature references. Conjugated ketones implicitly do not obey the octant rule. We discuss rules for interpreting the CD spectra of ˛,ˇ-and ˇ, - unsaturated ketones. This discussion is also followed by a graphical updating of their CD spectra. Finally, we attempt to update the reader on applications of the exciton chirality rule to ketones.
II. THE OCTANT RULE
The octant rule remains the most successful and longest serving chirality rule for interpreting ORD and CD spectra. It was formulated by Djerassi and colleagues3,16,17 more than 35 years ago and provided a way to determine (i) the absolute configuration of a saturated alkyl ketone or aldehyde when its conformation is known, or (ii) the conformation when the absolute configuration is known. It is doubtless the most important of the many chirality sector rules proposed for various chromophores10,18. Sector rules focus on the chromophore in a molecule and relate the CD spectrum to the chirality of the extra chromophoric environment, to the chirality of the chromophore, or to both19. The octant rule relates the n ! Ł CD spectrum of a saturated ketone or aldehyde to the extra chromophoric environment, to the molecule’s structure surrounding the CDO group. In chirality rules for unsaturated ketones, considerations of the chirality of the extended chromophore become important.
The octant rule is probably best summarized by the graphics of Figure 1. The ketone or aldehyde carbonyl chromophore is oriented along the Z axis while its oxygen and carbon and atoms conjoined lie in the YZ plane. The two local symmetry planes, XZ and YZ of the CDO, divide all surrounding space into quadrants, and a nonsymmetry-derived third nodal surface (A or B) divides quadrant space into octants. In the classical octant rule16, the third nodal surface was approximated as a plane (A). Subsequent theoretical and experimental studies defined it as a concave surface (B) in the revised octant rule20,21. To apply the octant rule, the observer looks down the CDO axis, from O to C. Front octants are nearer the observer; back octants are farther away, behind A or B. Groups or atoms lying on or near the octant surfaces make essentially no contribution to the octant rule and the CD of ketone or aldehyde. Groups or atoms lying off the octant surfaces make signed contributions according to the sign ascribed to each octant (Figure 1). These contributions are summed and weighted to predict the CD of the saturated ketone or aldehyde.
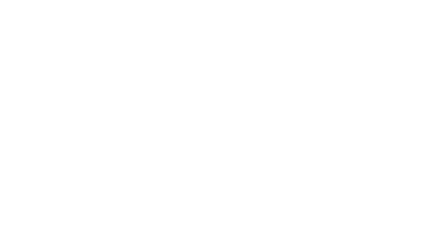
5. Chiroptical properties of compounds containing CDO groups |
157 |
FIGURE 1. (Left) Classical octant rule diagram (Reference 16) for the carbonyl n ! Ł transition of ketones and aldehydes. Local symmetry-derived, orthogonal octant planes XZ and YZ divide all space into quadrants, and a nonsymmetry-derived third nodal surface (A) is approximated by an orthogonal plane bisecting the CDO bond. To apply the octant rule, the observer looks down the CDO bond from O to C. ‘Front’ octants lie in the CZ direction and are those nearer and observer. ‘Back’ octants lie in theZ direction and are farther away from an observer. (Middle) Octant contribution signs from perturbers in back and in front octants. (Right) Revised octant rule (Reference 20) with octant planes XZ and YZ unchanged and the third nodal surface defined theoretically as a concave surface (B). Reprinted with permission from Reference 21. Copyright (1986) American Chemical Society
In order to use the octant rule to determine the absolute configuration of a ketone, its conformation must be known. With cyclohexanones, for example, one may assume a predominance of the chair conformation and orient the cyclohexanone so that the local symmetry planes of its carbonyl chromophore are coincident with those shown on the octant diagram of Figure 1. As illustrated in Figure 2, the preferred chair conformation of (3R)-methylcyclohexanone has an equatorial methyl. On the octant diagram, its methyl group lies in an upper left (C) back octant, whereas ring atoms 1, 2, 4 and 6 lie in symmetry planes and thus make no contributions. Carbons 3 and 5 lie in upper left (C) and upper right ( ) back octants, equally disposed across the XZ symmetry plane and thus make no net contribution. Consequently, the net contribution to the CD is positive, and a positive n ! Ł CD is predicted. Rotation of the molecule about the CDO (Z) axis by 180° would position the methyl group in a lower right (C) back octant, with the same conclusion. Strictly speaking, one weighs the contribution of the equatorial methyl carbon and its three hydrogens lying in the upper left (C) back octant vs an equatorial hydrogen lying in an upper right ( ) back octant. More ‘weight’ is given to the larger group, and hence a net positive n ! Ł CD Cotton effect is predicted. The experimentally observed CD is positive. If absolute configuration of 3-methylcyclohexanone had not been known, the observed positive Cotton effect would be interpreted by the octant rule to correspond to 3R. If the absolute configuration were known, the observed positive Cotton effect would fit best an equatorial chair conformation.
The possibility of ring conformational flexibility such as inversion (to afford the axial methyl isomer) or the intrusion of twist-boat isomers can be dismissed in (3R)- methylcyclohexanone because its n ! Ł Cotton effect sign and magnitude ( εmax284 C0.57)

158 |
Stefan E. Boiadjiev and David A. Lightner |
FIGURE 2. (a) Interconverting chair conformers of (3R)-methylcyclohexanone with axial (left) and equatorial (right) methyls. (b) CD and ORD spectra of (3R)-methylcyclohexanone showing positive CD and ORD Cotton effects. (c) Octant rule (Figure 1) applied to an octant projection diagram for (3R)-methylcyclohexanone with an equatorial methyl configuration. [(c) is modified from J. F. King, in
Elucidation of Structures by Physical and Chemical Methods, Part One (Ed. K. W. Bentley), Chap. VI, Wiley, New York, 1963 Reproduced by permission of John Wiley & Sons, Inc]
is very close to that observed of a rigid analog, (1S,3R)-4(e)-methyladamantan-2-one ( εmax284 C 0.67), where the cyclohexanone is locked into a chair conformation and the
methyl perturber is equatorial (Figure 3)21. Adamantanone is highly symmetric. All ring atoms lie on the XZ or YZ symmetry octant planes (Figure 1) or are equi-disposed across them. Only groups attached at ring carbons ˇ to the CDO make octant contributions. As such it is an ideal system for isolating and evaluating octant contributions. Significantly, replacing the methyl perturber with larger alkyl substituents (ethyl, isopropyl, tert-butyl or neohexyl) on the rigid and symmetric adamantanone framework causes relatively little change in the n ! Ł Cotton effect magnitude. These results indicated that the major octant contribution is made by the first carbon in the alkyl perturber chain, and the magnitude of octant contributions falls off rapidly with distance, as predicted much earlier3,16.
In general, octant contributions fall off with increasing distance of perturbers from the carbonyl chromophore. This is further illustrated by comparing the n ! Ł Cotton effects from ˛-axial methyl and ˇ-equatorial methyl perturbers on chair cyclohexanones as found in Table 1. Both methyl perturbers lie far from octant nodal planes (Figure 1), but the ˛-axial methyl lies closer to the carbonyl chromophore and thus makes a larger octant contribution. When the perturber lies close to an octant symmetry plane, as in an ˛- equatorial methylcyclohexanone, even if it also lies close to the carbonyl chromophore, the magnitude of the octant contribution is significantly diminished. With ˇ-methyls, neither the equatorial nor the axial lie near an octant symmetry plane. However, since the ˇ-axial lies closer to the carbonyl group than ˇ-equatorial, it was predicted16 to be an ordinary octant perturber with a strong positive n ! Ł Cotton effect. It was thus quite surprising when Snatzke and colleagues22 showed that a ˇ-axial methyl perturber not only did not
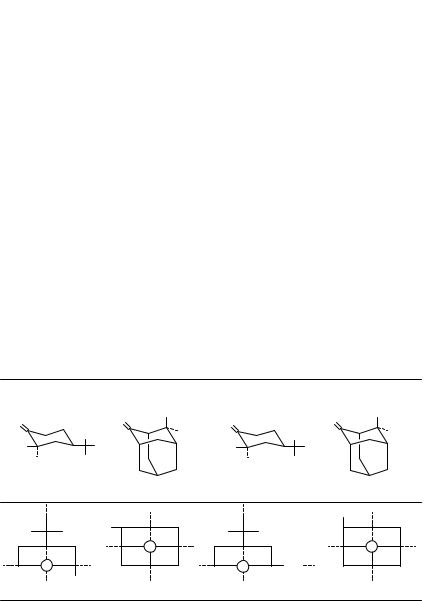
5. Chiroptical properties of compounds containing CDO groups |
159 |
FIGURE 3. (a) (1S,3R)-4(e)-Methyladamantan-2-one and its octant projection diagram. (b) (3R)- Methylcyclohexanone and its octant projection diagram. (c) CD spectra of (1S,3R)-4(e)-substituted
adamantan-2-ones in EPA (ether |
|
isopentane |
|
|
ethanol, 5:5:2, v/v/v) run at 25 °C and corrected to 100% |
||||||||||||||||||||||
|
|
||||||||||||||||||||||||||
e.e. The equatorial substituents are methyl (- - - - -), εmax |
. |
|
|
|
(- - |
Ð |
-), εmax |
D C |
0.81; |
||||||||||||||||||
|
|
|
(approx. |
- |
|
- |
|
-), ε |
max |
|
0.80; |
|
295 D C0 67; ethyl maxÐ |
|
|
295 |
|
||||||||||
isopropyl |
Ð |
Ð |
295 |
D C |
|
tert-butyl (approx. - |
Ð |
- |
Ð |
-), ε |
D C |
0.771; |
neohexyl |
||||||||||||||
max |
|
|
|
|
|
|
|
|
|
|
295 |
|
|
|
|||||||||||||
( |
|
), |
ε295 |
D C0.92. |
[(c) is reprinted |
with permission |
from |
|
Reference 21 |
Copyright (1986) |
|||||||||||||||||
|
|
American Chemical Society]
TABLE 1. Axial and equatorial ˛-methyl and ˇ-methylcyclohexanone units and their associated Cotton effects and octant diagrams
α-AxialCH3 |
β-EquatorialCH3 |
α-EquatorialCH3 |
|
β-AxialCH3 |
||
|
|
|
|
|
|
|
O |
O |
H |
O |
O |
CH3 |
|
CH3 |
H |
|||||
|
|
|
|
|||
H |
|
|
CH3 |
|
|
|
CH3 |
|
|
H |
|
|
|
|
|
|
|
|
|
|
∆ε − + 1.4 |
|
∆ε − + 0.6 |
∆ε − − 0.3 |
|
∆ε − − 0.1 |
|
~ |
|
~ |
~ |
|
~ |
• |
|
+ |
• |
+ • • |
• − |
H3 C • |
• |
• |
• |
|
• |
|
• |
|
• |
|
• |
• |
− |
|
CH |
− |
|
|
|
+ |
3 |
|
|
− |
|
• |
|
+ |
CH3 |
|
− |
• |
+ • |
• • |
− |
|
• |
• |
• |
• |
• |
• |
• |
|
• |
|
• |
• |
• |
|
• CH3 |
− |
• |
• |
• |
+ |
|
|
|
|
|
+ |
|
|
− |
|
+ |
|
|
|
|
obey the octant rule but also gave only a very weak Cotton effect, much smaller than ˛-equatorial. Since the ˇ-axial methyl does not lie near a CDO nodal plane (Figure 1), this observed ‘anti-octant’ behavior was astonishing. It was a source of much concern and led to a re-consideration of the octant rule that led to a better definition of the third nodal surface as concave (Figure 1)20,21.

160 |
Stefan E. Boiadjiev and David A. Lightner |
FIGURE 4. (a) Coordinate system for CDO group. (b) Relevant n-orbital of ketone carbonyl n ! Ł transition. The vertical XZ plane is a nodal plane for the n-orbital that bisects the R C R0 angle and lies perpendicular to the R(R0 )CDO local symmetry plane. (c) Relevant Ł -orbital of ketone n ! Ł transition. The horizontal YZ plane is a nodal plane for the Ł orbital and lies on the R(R0 )CDO local symmetry plane. (d) Carbonyl oxygen, as viewed looking down the Z-axis from O to C, showing circular movement of electron from the n to the Ł orbital
A. The Ketone C=O n ! pŁ Transition in Ketones and Aldehydes
The n ! Ł transition of ketones and aldehydes lies near 300 nm in the UV-visible spectrum. The relevant orbitals of n ! Ł transition are shown in Figure 4. The transition involves movement of an electron from an oxygen nonbonding (n) orbital (py ) to a Ł anti-bonding orbital comprising a linear combination of oxygen and carbon px orbitals. This rotation of charges leads to a large induced magnetic dipole moment ( E m D 1 Bohr magneton) oriented along the CDO bond (Z-axis), but it does not induce an electric dipole moment ( E e D 0) in the same direction. Therefore, on the basis of local symmetry, the n ! Ł transition is a magnetic dipole-allowed ( E m D6 0), electric dipole-forbidden ( E e D 0) transition. Thus, to a first approximation, the n ! Ł should not be observed. However, a weak UV absorbance (ε ' 10 100) near 300 nm is typically observed for ketones because electric dipole intensity is ‘borrowed’ through vibronic coupling from higher energy electric dipole-allowed transitions such as the ! Ł , which is polarized along the Z-axis (Figure 4). Although intensity borrowing provides a mechanism to observe n ! Ł absorption, it does not lead to optical activity of the chromophore, which still possesses a plane of symmetry. When the carbonyl group is located in a chiral environment, however, dissymmetric perturbations on the n ! Ł transition lead to a nonzero rotatory strength, R D E e Ð E m, and thus a nonzero CD.
B. C=O Symmetry Planes, Quadrants and The Third Nodal Surface
In the octant rule, all space surrounding the carbonyl chromophore is divided up into eight octants (Figure 1), and the octant occupied by a particular perturber determines the sign of its contribution to observed n ! Ł CD, to the rotatory strength of the n ! Ł transition. The octants are derived primarily from the local symmetry (C2v) of the carbonyl chromophore and the relevant orbitals of the n ! Ł transition. The two well-defined carbonyl symmetry planes (XZ and YZ, Figure 5a) coincide with the symmetry-derived nodal planes of the n and Ł electronic wavefunctions (Figure 4). They divide all space about the CDO group into quadrants. Quadrants are the minimum number of spatial subdivisions based on the intersecting symmetry planes of the isolated CDO chromophore. Using group theory, Schellman23 showed that a quadrant rule is the minimum sector rule for ketones. However, there may be additional nonsymmetry-derived nodal surfaces. Bouman and Moscowitz24 showed that if only n ! Ł and ! Ł states are mixed by incomplete coulombic screening, a quadrant rule is obtained for a localized n-orbital. However, if the n-orbital is delocalized, an incorrect octant rule is predicted. On the
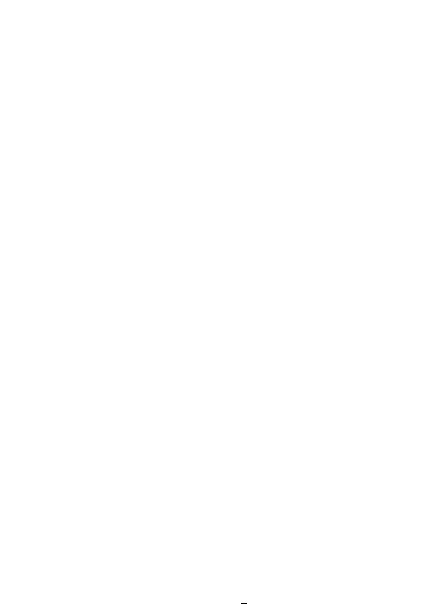
5. Chiroptical properties of compounds containing CDO groups |
161 |
FIGURE 5. (a) CDO symmetry (C2v) planes that divide all space surrounding the carbonyl group into quadrants. The C or signed contributions that perturbers make are denoted: upper right and lower left, negative; lower right and upper left, positive. (b) The third nodal surface associated with the Ł orbital and approximated by a plane (A) that lies midway between the C and O. (c) Concave third nodal surface (B) derived by theory and experiment
other hand, mixing of the n ! Ł state with D states generated from 3d-orbitals leads to the octant rule behavior with the correct sign for hydrogen and carbon perturbers. In either case, the contribution to the CD intensity from the D states is in most instances about an order of magnitude greater than that obtained from mixing with the ! Ł states. Thus, whatever ‘quadrant’ contributions come into play are suppressed by ‘octant’ contributions.
Moffitt and colleagues16 took the third surface to be a plane bisecting the CDO bond (Figure 5b) purely for convenience rather than on any theoretical basis. Indeed they specifically cautioned that this surface was very probably not a plane. Subsequently, Bouman and Lightner20 showed by theory and experiment that the shape of the third nodal surface is closer to concave, cutting behind the carbonyl carbon (Figure 5c).
Reflection of a perturber across either of the symmetry planes (XZ and YZ, Figures 4 and 5) leads to a mirror image molecular fragment, and hence one with oppositely-signed rotatory strength. Since the third nodal surface does not follow from symmetry, ‘reflection’ across it does not correspond to a mirror image situation, and hence the weight given to a perturber in a front octant is not the same as for a like position in a back octant. Each atom or group surrounding the CDO chromophore makes a signed contribution to the observed n ! Ł CD. The signs for atoms such as C, H, Cl, Br and I are shown in Figure 5. Atoms lying in symmetry planes offer no contribution, and atoms symmetrically located across the carbonyl symmetry planes will exert no effect on the CD, due to cancellation. In general, the sign made by an octant perturber to the observed CD of the n ! Ł transition varies as the sign of the product, X Ð Y Ð Z of the atomic coordinates, as defined in Figures 1, 4 and 5. Contributions are assumed to be additive, and the magnitudes of the contributions vary according to the nature of the perturber while falling off rapidly with increasing distance from the carbonyl chromophore or closeness to the nodal surfaces. Broadly speaking, the octant rule, a geometrical rule so simple and straightforward to apply, has served well in establishing the absolute configuration and in elucidating the conformation of a large number of compounds3 20.
C. Front Octants and Anti-Octant Effects
The validity of the octant rule is supported largely and convincingly by ORD and CD spectra from numerous examples where the dissymmetric perturbers (the groups perturbing the carbonyl chromophore in a nonsymmetric way), such as the methyl group of
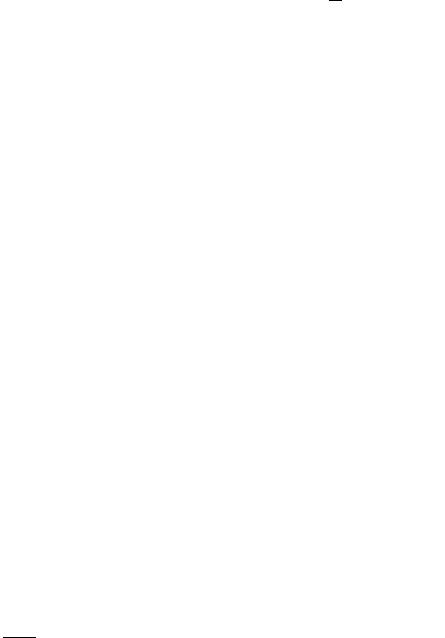
162 Stefan E. Boiadjiev and David A. Lightner
3(R)-methylcyclohexanone, are invariably located in back octants behind the carbon of the carbonyl group. There are extremely few examples in which dissymmetric perturbers are found in front of the carbonyl carbon or oxygen. And in most of these few examples there are also dissymmetric perturbers in back octants. Although there was some doubt over the existence of or need for front octants, it was quite obvious at an early stage in the development of the octant rule that ketones could be found where some atoms would lie in front octants, e.g. in 1-oxo, 7-oxo- and 11-oxosteroids. Here, however, octant contributions from atoms lying in back octants always appeared to dominate the sign of the Cotton effect3,16. Examples of contributors entering front octants were discussed by Djerassi and Klyne25, but their examples also had back octant as well as front octant contributions and thus did not clearly test the existence of front octants. Kirk, Klyne and Mose26 prepared structurally related D-homo and D-nor 7-ketosteroids and subtracted Cotton effect of the D-norsteroid from that of the D-homosteroid to estimate its front octant contributions. The authors concluded in favor of an octant rather than a quadrant rule. At the same time, CD spectra of potentially cleaner examples, cis- and trans-6-methylspiro[4.4]nonan-1- ones, supported the notion of front octants, but the analysis was complicated by ring conformational changes27. With the lack of unambiguous proof for the existence of front octants, the octant rule remained incompletely proved by experiment until 1974, when the existence of front octants was shown unequivocally by the synthesis and CD28 of two spiroketones, syn-(10R)-spiro[cyclobutan-2-one-1,20-(40(a)-methyladamantane)] and its anti isomer (Figure 6), prepared from ( )-(1R,3S)-4(R)(a)-methyladamantan-2-one (Table 1). In the former the methyl group lies in front of the carbonyl oxygen; in the
FIGURE 6. (Left) CD spectra of syn-(10 R)-spiro[cyclobutan-2-one-1,20 -(40 (a)-methyladamantane)] ( ) anti-(10 R)-spiro[cyclobutan-2-one-1,20 -(40 (a)-methyladamantane (- - - - -) in isopentane at 20°. (Right) CD spectra of syn-(10 R)-spiro[cyclobutan-2-one-1,70 -(20 -exo-methylnorbornane)] in isopentane at 20 °C Corrections are made to 100% optical purity. [(Right) Reproduced by permission of The Royal Society of Chemistry from Reference 29; (Left) Reprinted with permission from Reference 28. Copyright (1974) American Chemical Society]
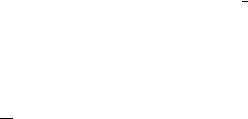
5. Chiroptical properties of compounds containing CDO groups |
163 |
latter it lies well behind. With a time-average planar cyclobutanone conformation, the plane of the cyclobutanone ring bisects the spiro-fused adamantane skeleton. That is, in the absence of the methyl group, the plane of the cyclobutanone ring would lie on an octant symmetry plane (YZ, Figure 1). Thus, the methyl group, which does not lie on a symmetry or nodal plane, represents the lone dissymmetric perturber of the ketone carbonyl chromophore. In a quadrant rule, the methyl perturbers of both spiro-adamantyl cyclobutanones would lie in oppositely-signed quadrants: upper left or lower right positive for the syn and upper right or lower left negative for the anti (Figure 5a). However, the observed n ! Ł CD Cotton effect signs are the same for the syn and the anti. The negative sign of the anti is in agreement with both a quadrant rule and the octant rule, but the negative sign of the syn is in agreement only with the octant rule. The methyl group of the syn lies in an upper left or lower right ( ) front octant. Additional support for front octants comes from the positive n ! Ł Cotton effect of spiro-bornylcyclobutanone29 (Figure 6b), which has a different carbocyclic framework, but one where the lone dissymmetric methyl perturber lies in front of the carbonyl oxygen in an upper right or lower left positive front octant. Even considering puckering of the cyclobutanone ring and attendant changes in octant locations of the perturbers, the n ! Ł Cotton effect is still dominated by a methyl perturber in a front octant30. The syn spiroketones of Figure 6 thus provided the previously missing unequivocal experimental proof for the existence of front octants.
Instances where octant rule behavior was predicted and not observed, the problem of ‘anti-octant’ effects, emerged shortly after the octant rule was first postulated3,16, e.g. in a theoretical derivation of the octant rule in 1966, where Pao and Santry31 calculated the n ! Ł Cotton effects of various methyl-substituted chair cyclohexanones. Their results from Gaussian orbital calculations for all methyl configurations except 3-axial agreed with those predicted by Moscowitz in his theoretical derivation of the octant rule32. Later calculations using an extended Huckel¨ treatment33 agreed with the new prediction for axial 3-methylcyclohexanone. (It is important to note that Moscowitz did not include this structure in his calculations.) These findings suggested that the third nodal surface might be curved. At nearly the same time, Snatzke and coworkers34,35 published the first experimental verification that the 3-axial substituents on chair cyclohexanone gave antioctant contributions to the octant rule. (1R,3S)-4(R)(a)-methyladamantan-2-one (mirror image shown in Table 1) gave a weak, positive CD Cotton effect in ethanol or dioxane solvent36, opposite to the predicted negative Cotton effect for the methyl perturber in a lower left or upper right back octant. The significance of this surprising observation was obscured by the fact that a weak negative Cotton effect was seen when the solvent was changed to isooctane for the same ketone. Yet adamantanones with other ˇ-axial perturbers were found to exhibit ‘anti-octant’ CD Cotton effects which did not change sign: Cl, Br, I, N322,36 and SCN, ONO2, OAc, OCO2CH335.
Other apparent ‘anti-octant’ effects were found shortly thereafter37 41. The most perceptive of these is the work of Coulombeau and Rassat37, who analyzed CD and ORD data for a number of ketones and made the proposal that the third nodal surface was convex, curving sharply away from the carbonyl oxygen. They thus explained ‘anti-octant’ behavior in terms of the perturber actually lying in front of the third nodal surface as they defined it. Tocanne drew a similar conclusion from his cyclopropylketone work41. Although other anti-octant effects had been noted prior to 197442,43, at that time there was no unambiguous experimental demonstration from carbon and hydrogen as static, dissymmetric perturbers except the work of Snatzke and Eckhardt36 in which a peculiar solvent effect had been noted. In 1974 it was shown that the stereochemically rigid and well-defined (1R)-exo-2-methyl-7-norbornanone and (1R)-endo-2-methyl-7-norbornanone gave negative and positive n ! Ł CD Cotton effects, respectively, in isopentane42
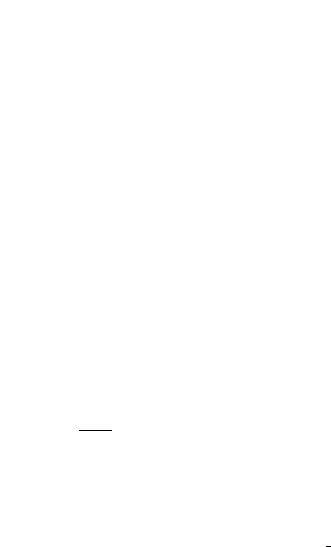
164 |
Stefan E. Boiadjiev and David A. Lightner |
FIGURE 7. CD spectra of (1R)-exo-2-methylbicyclo[2.2.1]heptan-7-one (- - - - -) and (1R)-endo-2- methylbicyclo[2.2.1]heptan-7-one ( ) in isopentane at 20 °C Corrections are made to 100% optical purity. [Reprinted with permission from Reference 42. Copyright (1974) American Chemical Society]
(Figure 7). Since 7-norbornanone is achiral, the methyl perturbers represent the lone dissymmetric octant perturbers, which in both compounds lie behind the third nodal surface (A, Figure 1) in a positive upper left or lower right octant. It thus appeared that the apparent ‘anti-octant’ effect should not be interpreted as such but rather as a front octant effect, with the exo methyl perturber lying in a negative front octant. And it was becoming likely that other so-named ‘anti-octant’ effects for alkyl and other perturbers36 38 could be attributed to the perturbers lying in front octants42.
In 1976, Bouman and Lightner20, in a detailed theoretical analysis and CNDO/S calculations of the known ‘anti-octant’ compounds and a series of decalones, updated the octant rule by defining the third, nonsymmetry-derived nodal surface as concave (from the perspective of a viewer looking down the CDO bond, from O to C, as in B of Figure 1). The third nodal surface thus bends outward in the CZ direction. Although this might appear to contradict the empirical results, surface B cuts just behind the 3-axial position, which locates the ‘anti-octant’ ˇ-axial methyl group of adamantanone and the 2-exo-methyl group of 7-norbornanone in front octants. Replacing the methyl perturber with larger groups that project even farther into front octants, as in (1S,3R)-4(a)-ethyl or