
Radiation Physics for Medical Physiscists - E.B. Podgorsak
.pdf1.25 Tunneling |
39 |
ever, in certain experiments such as in Compton e ect the photon behaves like a particle; in other experiments such as double-slit di raction it behaves like a wave.
Another example of the particle-wave duality are the wave-like properties of electrons as well as heavy charged particles and neutrons that manifest themselves through di raction experiments (see, for example, DavissonGermer experiment discussed in Sect. 1.21).
1.25 Tunneling
The particle-wave duality is highlighted in discussions of potential wells and potential barriers in quantum and wave mechanics; the potential wells attract and trap particles, potential barriers reflect or transmit them. While medical physics and clinical physics rarely deal with quantum and wave mechanics, there are several physical phenomena of importance to radiation physics and, by extension, to medical physics that can only be explained through wavemechanical reasoning. Tunneling, for example, is a purely wave-mechanical phenomenon that is used in explaining two important e ects in radiation physics: α decay and field emission. In addition, there are several other phenomena of importance in electronics that can be explained invoking tunneling such as, for example, in the periodic inversion of the ammonia molecule NH3, used as standard in atomic clocks, and in a semiconductor device called tunnel diode that is used for fast switching in electronic circuits.
A classical particle incident on a square barrier will pass the barrier only if its kinetic energy EK exceeds the barrier potential EP. If EP > EK, the classical particle is reflected at the barrier and no transmission occurs because the region inside the barrier is forbidden to the classical particle.
A quantum-mechanical particle incident on a square barrier has access to regions on both sides of the barrier, irrespective of the relative magnitudes of the kinetic energy EK and the barrier potential EP. A matter wave is associated with the particle and it has a non-zero magnitude on both sides of the barrier as well as inside the barrier. The wave penetrates and traverses the barrier even when EP > EK, clearly contravening classical physics but conforming to the rules of wave mechanics. The wave function associated with a quantum-mechanical particle incident on a barrier must be continuous at the barrier, will exhibit an exponential decay inside the barrier, and will be continuous on the far side of the barrier. The non-zero probability for finding the particle on the opposite side of the barrier indicates that the particle may tunnel through the barrier or one may say that the particle undergoes the tunneling e ect. In tunneling through a barrier, the particle behaves as a pure wave inside the barrier and as a pure particle outside the barrier.
40 1 Introduction to Modern Physics
1.25.1 Alpha Decay Tunneling
Alpha decay is considered a tunneling phenomenon in which α particles with kinetic energies between 4 MeV and 9 MeV tunnel through a potential barrier of the order of 30 MeV. The tunneling theory of the α decay was proposed by George Gamow in 1928. Inside the parent nucleus (atomic number Z) the α particle is free yet confined to the nuclear potential well by the strong nuclear force. The dimension of the well is of the order of few fm; once the α particle is beyond this distance from the center of the parent nucleus, it only experiences Coulomb repulsion between its charge 2e and the charge of the daughter nucleus (Z − 2)e.
A classical α particle with kinetic energy EK < 9 MeV cannot overcome a potential barrier with EP > 30 MeV. On the other hand, a α particle with wave-like attributes may tunnel through the potential barrier and escape the parent nucleus through this purely quantum-mechanical phenomenon.
1.25.2 Field Emission Tunneling
Field emission is another interesting e ect that is explained by the tunneling phenomenon. Here one relies on the wave phenomena of electrons to explain how electrons tunnel through a potential barrier (of the order of the work function) to escape the atoms of a metal through the application of a very strong electric field. This e ect may be considered cold cathode emission in contrast to the well-known heated cathode emission that is used in the socalled Coolidge x-ray tubes, electron guns in linear accelerators, and many other instruments relying on the hot filament sources where electrons get “boiled-o ” from the heated cathode. Modern application of field emission is in field emission microscope and in scanning tunneling microscope.
1.26 Maxwell’s Equations
The basic laws of electricity and magnetism can be summarized by four basic Maxwell’s equations on the assumption that no dielectric or magnetic material is present. Each of the four basic Maxwell’s equations is also known under its own specific name honoring the physicist who derived the equation independently before Maxwell unified electric and magnetic forces under one umbrella. In radiation physics and in medical physics Maxwell’s equations play an important role in the understanding of bremsstrahlung production, in waveguide theory of particle acceleration, and in the theory of ionization chamber operation.
Maxwell’s equations may be expressed in integral form or in di erential form and the two forms are linked through two theorems of vector calculus: the Gauss’s divergence theorem and the Stokes’s theorem. For a vector func-
tion A and volume V bounded by a surface S the two theorems are given as follows:

|
|
|
|
|
1.26 |
Maxwell’s Equations |
41 |
|
Gauss’s theorem |
|
|
|
|
|
|
||
V |
|
|
|
|
|
dS |
(1.85) |
|
|
A dV = |
divA dV = A |
|
|||||
· |
V |
|
S |
· |
|
|
||
Stokes’s theorem |
|
|
|
|
|
|
||
S |
( × A) · dS = S |
curlA · dS = |
A · d |
(1.86) |
Maxwell’s equations (integral form on the left, di erential form on the right) are given as follows:
|
|
|
|
|
o |
|
|
|
|
|
|
· E |
|
|
|
o |
|
|
|
|
|||
|
|
|
|
q |
|
|
|
|
|
|
|
|
|
|
ρ |
|
|
|
|
||||
E · dS = |
ε |
|
|
|
|
|
|
|
|
|
|
|
= |
ε |
|
|
|
|
|
Gauss’s law |
|||
|
|
|
|
|
|
|
|
|
|
|
|
|
· B |
|
|
|
|
|
|
(1.87) |
|||
|
|
|
|
|
|
|
|
|
|
|
|
|
|
|
|
|
|
|
|
||||
|
|
|
|
|
|
|
|
|
|
|
|
|
|
|
= 0 |
|
|
|
|
Gauss’s law |
|||
B |
· dS = 0 |
|
|
|
|
|
|
|
|
|
|
|
|
|
|||||||||
|
|
|
|
|
|
|
|
|
|
|
|
|
|
|
|
|
|
|
|
|
|
(1.88) |
|
E · |
|
|
− |
|
|
∂t |
|
B · |
|
|
× E |
− |
|
|
|
|
|||||||
|
|
|
|
|
|
|
∂t |
|
|
||||||||||||||
|
|
d = |
|
|
|
∂ |
|
|
|
dS |
|
|
= |
|
|
|
∂B |
|
Faraday’s law |
||||
|
|
|
|
|
|
|
|
|
|
|
|||||||||||||
|
|
|
|
|
|
|
|
|
|
|
|
|
|
|
|
|
|
|
|
|
|
(1.89) |
|
B · |
|
o |
|
|
c2 |
|
∂t |
E · |
|
× B |
|
|
|
o |
|
|
|
||||||
|
|
|
|
|
|
|
|
|
∂t |
||||||||||||||
|
|
d =µ |
|
I + |
1 |
|
∂ |
|
|
dS) |
|
|
= µ |
|
(j + |
∂B |
) Amp`ere’s law, |
||||||
|
|
|
|
|
|
|
|
||||||||||||||||
|
|
|
|
|
|
|
|
|
|
|
|
|
|
|
|
|
|
|
|
|
|
(1.90) |
|
|
|
|
|
|
|
|
|
|
|
|
|
|
|
|
|
|
|
|
|
|
|
||
where E and |
|
B are the electric and magnetic fields, respectively; ρ and j |
are the charge density and current density, respectively, εo and µo are the permittivity and permeability, respectively, of vacuum; q is the total charge; and I is the current.
Maxwell’s equations combined with the Lorentz force and Newton’s second law of motion provide a complete description of the classical dynamics of interacting charged particles and electromagnetic fields. The Lorentz force
|
|
|
FL for charge q moving in electric field E and magnetic field B with velocity |
||
υ is given as follows: |
|
|
|
|
(1.91) |
FL = q(E + υ × B) . |
Ernest Rutherford and Niels Bohr, Giants of Modern Physics
The photographs on the next page show stamps issued in honor of physicists Ernest Rutherford (1871–1937) and Niels Bohr (1885–1962), the two scientists credited with developing our current atomic model. According to the Rutherford-Bohr atomic model most of the atomic mass is concentrated in the positively charged nucleus and the negative electrons revolve in orbits about the nucleus. New Zealand issued the stamp for Rutherford and Denmark for Bohr, both countries honoring their respective native son. Both physicists received Nobel Prizes for their work: Rutherford in 1908 “for investigations into the disintegration of elements and the chemistry of radioactive substances”, Bohr in 1922 for work on the structure of atoms.
Rutherford studied in New Zealand and England, but spent all his professional life first in Canada at McGill University in Montreal (1898–1907) and then in England at the University of Manchester (1908–1919) and the Cavendish Laboratory in Cambridge (1919–1937). During his nine years at McGill, Rutherford published some 70 papers and worked with Frederick Soddy on the disintegration theory of radioactivity. In his 12 years at the University of Manchester, Rutherford collaborated with Geiger and Marsden and, based on their experiments, proposed the nuclear model of the atom. In Cambridge, Rutherford continued using alpha particles from radium and polonium sources to probe the atom. He collaborated with James Chadwick who in 1932 discovered the neutron and with Charles Wilson, the developer of the “cloud chamber”.
Rutherford is considered one of the most illustrious scientists of all time. His work on the atomic structure parallels Newton’s work in mechanics, Darwin’s work on evolution, Faraday’s work in electricity and Einstein’s work on relativity.
Bohr studied at the Copenhagen University and spent most of his professional life there, except for short intervals in 1912 and in 1914–1916 when he worked with Rutherford in Manchester. He built a renowned school of theoretical and experimental physics at the University of Copenhagen and became its first director from its inauguration in 1921 to his death in 1962. The school is now known as the Niels Bohr Institute. Bohr is best known for his introduction of the electron angular momentum quantization into the atomic model that is now referred to as the Rutherford-Bohr atom. He also made numerous other contributions to theoretical physics, most notably with his complementarity principle and the liquid drop nuclear model.
Bohr was also interested in national and international politics and advised Presidents Roosevelt and Truman as well as Prime Minister Churchill on scientific issues in general and nuclear matters in particular. Bohr enjoyed a tremendous esteem from physics colleagues, world leaders and the general public, and among the scientists of the 20th century only Albert Einstein and Marie Curie have reached the same level.
c New Zealand Post (1999); Stamp depicting Ernest Rutherford. Reproduced with Permission.
c Post Danmark (1963) and Sven Bang; Stamp depicting Niels Bohr. Designed by Viggo Bang and Reproduced with Permission.
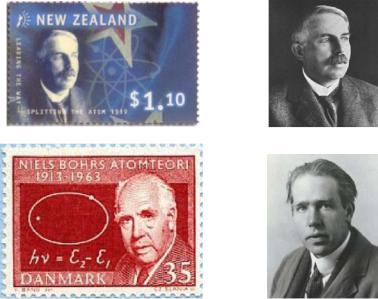
2 Rutherford–Bohr Atomic Model
This chapter is devoted to a discussion of the Rutherford–Bohr atomic model. First, it covers the intriguing Geiger–Marsden experiment of α-particle scattering on thin gold foils, the results of which lead to the Rutherford’s ingenious conclusion that most of the atom is empty space and that most of the atomic mass is concentrated in an atomic nucleus. The kinematics of α-particle scattering is then discussed in some detail and the Bohr derivation of the hydrogen atom kinematics is presented. The simple hydrogen atom calculations are supplemented with corrections for the finite mass of the nucleus and the correspondence principle is introduced. Next, the concepts of one-electron atoms are expanded to multi-electron atoms through the Hartree approximation, and the exclusion principle and the periodic table of elements are introduced. The chapter concludes with a description of experiments that substantiate the Rutherford–Bohr atomic model and with a calculation of the ground state of the hydrogen atom based on the timeindependent Schr¨odinger equation.
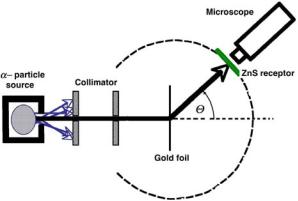
44 2 Rutherford–Bohr Atomic Model
2.1 Geiger–Marsden Experiment
Hans Geiger and Ernest Marsden in 1909 carried out an experiment studying the scattering of 5.5 MeV α particles on a thin gold foil with a thickness of the order of 10−6 m. The α particles were obtained from radon-222, a natural α-particle emitter. The experiment, shown schematically in Fig. 2.1, seems rather mundane, however, its peculiar and unexpected results had a profound e ect on modern physics in particular and on the humanity in general.
At the time of the Geiger–Marsden experiment Thomson’s atomic model was the prevailing atomic model based on an assumption that the positive and the negative (electron) charges of an atom were distributed uniformly over the atomic volume (“plum-pudding” model).
It was Ernest Rutherford who concluded that the peculiar results of the Geiger–Marsden experiment did not support the Thomson’s atomic model and proposed the currently accepted model in which:
1.mass and positive charge of the atom are concentrated in the nucleus the size of which is of the order of 10−15 m;
2.negatively charged electrons revolve about the nucleus.
The two competing atomic models are shown schematically in Fig. 2.2 (see Sect. 2.2.1); the Thomson model in part a, the Rutherford model in part b.
2.1.1 Parameters of the Geiger–Marsden Experiment
In the Geiger–Marsden experiment the kinetic energy of the α particles was 5.5 MeV and the radius of the gold–79 nucleus 19779 Au is determined as follows [see (1.14)]:
√ |
|
|
√ |
|
|
|
|
3 |
A = 1.4 fm |
3 |
197 |
≈ 8 fm . |
(2.1) |
||
R = Ro |
|
Fig. 2.1. Schematic diagram of the Geiger–Marsden experiment in the study of α-particle scattering on gold nuclei in a thin gold foil. Θ is the scattering angle

2.1 Geiger–Marsden Experiment |
45 |
The distance of closest approach Dα−N between the α particle and the nucleus is determined by equating EK, the kinetic energy of the α particle at ∞, with EP, the electric potential energy at Dα−N, and solving for Dα−N to get
D |
α−N |
= zZe2 1 = |
2 × 79 × e × 1.6 × 10−19 As Vm |
|||||
|
|
4πεo |
|
EK |
|
4π × 8.85 × 10−12 As 5.5 × 106 eV |
|
|
|
|
≈ 41 fm. |
(2.2) |
Three important observations may now be made based on these parameters:
•For naturally occurring α particles interacting with nuclei of atoms the distance of closest approach Dα−N exceeds the radius R of the nucleus. The α particle thus does not penetrate the nucleus.
•Nature provided Geiger and Marsden with ideal conditions to probe the nucleus. The radon-222 α particles have an energy of 5.5 MeV which is large enough to allow penetration of the atom but it is neither too large to cause nuclear penetration with associated nuclear reactions nor is it too large to require relativistic treatment of the α-particle velocity.
•Since artificial nuclear reactions and the relativistic mechanics were not understood at the time when the Geiger–Marsden experiment was carried out, Rutherford would not be able to solve with such elegance the atomic model question if the kinetic energy of the α particles used in the experiment was much larger or much smaller than 5.5 MeV.
The speed υα of the α particles relative to the speed of light in vacuum c for 5.5 MeV α particles can be calculated using either the classical or the relativistic relationship (note that mαc2 ≈ 4 × 939 MeV):
The classical calculation is done using the classical expression for the
kinetic energy EK of the α particle [see (1.45)] |
|
||||||||||||||||
|
|
mαυ2 |
1 |
|
|
|
|
υα2 |
|
||||||||
EK = |
|
|
|
α |
= |
|
mαc2 |
|
|
. |
|
(2.3) |
|||||
|
2 |
2 |
c2 |
||||||||||||||
Solve (2.3) for υα/c to obtain |
|
|
|
|
|
|
|
||||||||||
|
|
|
|
|
|
= |
|
|
|
|
|
||||||
|
c = |
|
mαc2 |
4 |
× |
939 MeV = 0.054 |
(2.4) |
||||||||||
υα |
|
|
|
2EK |
|
|
|
2 |
|
|
5.5 M eV |
|
|||||
|
|
|
|
|
|
|
|
|
|
|
× |
|
|
|
|
|
The relativistic calculation using the relativistic expression for the kinetic energy EK of the α particle
EK = |
|
mαc2 |
|
|
− mαc2 . |
(2.5) |
|
|
|
|
|
|
|||
1 − |
υα |
|
2 |
||||
|
c |
|
|
|
Solve (2.5) for υα/c to obtain
υα |
|
|
|
|
1 |
|
|
|
|
|
1 |
|
|
|
||
c = |
1 − |
|
1 + mα c2 |
|
2 = |
1 + 4 939 |
|
2 = 0.054 . (2.6) |
||||||||
|
|
|
|
|
|
|
|
|
|
|
× |
|
|
|
||
|
|
|
|
|
|
|
|
|
|
|
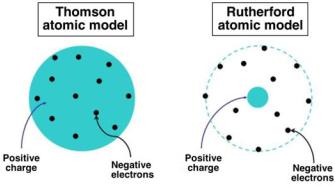
46 2 Rutherford–Bohr Atomic Model
The relativistic and classical calculations give identical results since the ratio of velocities (υα/c) 1 for particles with kinetic energy EK of the order of a few MeV. Rutherford’s use of the simple classical relationship for the kinetic energy of the naturally occurring α particles was thus justified.
2.1.2 Thomson’s Atomic Model
Joseph J. Thomson, who is also credited with the discovery of the electron in 1897, proposed an atomic model in which the negatively charged electrons were dispersed uniformly within a continuous spherical distribution of posi-
˚
tive charge with a radius of the order of 1 A. The electrons formed rings and each ring could accommodate a certain upper limit in the number of electrons and then other rings began to form. With this ring structure Thomson could in principle account for the periodicity of chemical properties of elements. A schematic representation of the Thomson’s atomic model (“plum-pudding model ”) is given in Fig. 2.2a.
–In the ground state of the atom the electrons are fixed at their equilibrium positions and emit no radiation.
–In an excited state of the atom the electrons oscillate about their equilibrium positions and emit radiation through dipole oscillations by virtue of possessing charge and being continuously accelerated or decelerated (Larmor relationship).
According to the Thomson’s atomic model the angular distribution of α particles scattered in the gold foil in the Geiger–Marsden experiment is
Fig. 2.2. Schematic diagram of two atomic models: a Thomson’s “plum-pudding” model in which the electrons are uniformly distributed in the sea of positive atomic charge and b Rutherford’s nuclear model in which the electrons revolve in empty space around the nucleus that is positively charged and contains most of the atomic mass

|
|
2.2 Rutherford Atom and Rutherford Scattering |
47 |
|||||
Gaussian and given by the following expression |
|
|||||||
|
2IΘ |
|
|
|
|
|||
N (Θ)dΘ = |
e−Θ2/Θ2 |
dΘ , |
(2.7) |
|||||
|
|
|
||||||
|
|
Θ2 |
|
where
Θis the scattering angle of the α particle after it passes through the gold foil (the α particle undergoes 104 interactions as a result of a foil thickness of 10−6 m and an approximate atomic diameter of 10−10 m),
N (Θ)dΘ is the number of α particles scattered within the angular range of Θ to Θ + dΘ,
Iis the number of α particles striking the gold foil,
Θ2 |
is the mean square net deflection experimentally determined to be |
||
|
of the order of 3 × 10−4 rad2, i.e., ( |
|
)1/2 = 1o. |
|
Θ2 |
Geiger and Marsden found that more than 99% of the α particles incident on the gold foil were scattered at angles less than 3◦ and that their distribution followed a Gaussian distribution given in (2.7). However, Geiger and Marsden also found that one in 104 α particles was scattered with a scattering angle Θ > 90◦ which implied a probability of 10−4 for scattering with Θ > 90◦, in drastic disagreement with the probability of 10−3500 predicted by the theory based on the Thomson’s atomic model, as shown below.
According to the Thomson atomic model the probability for α-particle scattering with Θ > 90◦ (i.e., with a scattering angle Θ between π/2 and π) is calculated by integrating (2.7) as follows:
N (Θ > 2 ) = |
π |
|
|
= |
|
e−Θ /Θ2 d(Θ2/Θ2) = e− Θ2 |
|
|||||||||||
π |
|
|
|
|
||||||||||||||
π |
N (Θ)dΘ |
|
π |
|
|
|
|
|
|
|
|
|
|
2 |
π |
|||
I |
I |
|
|
|
− |
|
|
|
|
|
|
|
|
− |
|
|
π/2 |
|
|
|
/2 |
|
|
|
π/2 |
|
|
2 |
|
|
|
|
|
|
Θ |
|
|
|
|
|
|
|
|
|
|
|
|
|
|
|
|
|
|
|
||
|
|
|
|
|
|
|
|
|
|
|
|
|
|
|
|
|
|
|
|
|
180o |
} |
2 |
|
90o |
} |
2 |
= e−90 |
2 |
≈ 10−3500 , |
|
|
|
||||
|
= −e−{ 1o |
+ e−{ 1o |
|
|
|
|
(2.8) |
where we use the value of 1◦ for the root mean square angle Θ2.
2.2 Rutherford Atom and Rutherford Scattering
The theoretical result of 10−3500 for the probability of α-particle scattering with a scattering angle greater than 90◦ on a Thomson atom is an extremely small number in comparison with the result of 10−4 obtained experimentally by Geiger and Marsden. This highlighted a serious problem with the Thomson’s atomic model and stimulated Ernest Rutherford to propose a completely new model that agreed with experimental results obtained by Geiger and Marsden.

48 2 Rutherford–Bohr Atomic Model
2.2.1 Rutherford Model of the Atom
Contrary to Thomson’s “plum-pudding” atomic model, depicted schematically in Fig. 2.2a, essentially all mass of the Rutherford atom is concentrated in the atomic nucleus that is also the seat of the positive charge of the atom and has a radius of the order of 10−15 m, as shown schematically in Fig. 2.2b. Electrons are distributed in a spherical cloud on the periphery of the Rutherford atom with a radius of the order of 10−10 m.
2.2.2 Kinematics of Rutherford Scattering
Based on his model and four additional assumptions, Rutherford derived the kinematics for the scattering of α particles on gold nuclei using basic principles of classical mechanics. The four additional assumptions are as follows.
1.the mass M of the gold nucleus is much larger than the mass of the α particle mα, i.e., M mα.
2.Scattering of α particles on atomic electrons is negligible because mα me, where me is the electron mass.
3.The α particle does not penetrate the nucleus (no nuclear reactions).
4.The classical relationship for the kinetic energy EK of the α particle, i.e., EK = mαυ2/2, is valid, where υ is the velocity of the α particle.
The interaction between the α particle (charge ze) and the nucleus (charge Ze) is a repulsive Coulomb interaction between two positive point charges, and, as a result, the α particle follows a hyperbolic trajectory, as shown schematically in Fig. 2.3.
As shown in Fig. 2.3, the nucleus is in the outer focus of the hyperbola because of the repulsive interaction between the α particle and the nucleus. The relationship between the impact parameter b and the scattering angle θ may be derived most elegantly by determining two independent expressions for the change in momentum ∆p of the scattered α particle. Note that θ represents the scattering angle in a single α-particle interaction with one nucleus, whereas Θ represents the scattering angle resulting from the α particle traversing the thin gold foil and undergoing 104 interactions while traversing the foil.
The momentum transfer is along a line that bisects the angle π − θ, as indicated in Fig. 2.3. The magnitude of the Coulomb force Fcoul acting on the α particle is given by
Fcoul = |
zZe2 1 |
, |
(2.9) |
4πεo r2 |
where
ris the distance between the α particle and the nucleus M ,
z is the atomic number of the α particle (for helium z = 2 and A = 4), Z is the atomic number of the absorber (for gold Z = 79 and A = 197).