
- •Preface
- •Imaging Microscopic Features
- •Measuring the Crystal Structure
- •References
- •Contents
- •1.4 Simulating the Effects of Elastic Scattering: Monte Carlo Calculations
- •What Are the Main Features of the Beam Electron Interaction Volume?
- •How Does the Interaction Volume Change with Composition?
- •How Does the Interaction Volume Change with Incident Beam Energy?
- •How Does the Interaction Volume Change with Specimen Tilt?
- •1.5 A Range Equation To Estimate the Size of the Interaction Volume
- •References
- •2: Backscattered Electrons
- •2.1 Origin
- •2.2.1 BSE Response to Specimen Composition (η vs. Atomic Number, Z)
- •SEM Image Contrast with BSE: “Atomic Number Contrast”
- •SEM Image Contrast: “BSE Topographic Contrast—Number Effects”
- •2.2.3 Angular Distribution of Backscattering
- •Beam Incident at an Acute Angle to the Specimen Surface (Specimen Tilt > 0°)
- •SEM Image Contrast: “BSE Topographic Contrast—Trajectory Effects”
- •2.2.4 Spatial Distribution of Backscattering
- •Depth Distribution of Backscattering
- •Radial Distribution of Backscattered Electrons
- •2.3 Summary
- •References
- •3: Secondary Electrons
- •3.1 Origin
- •3.2 Energy Distribution
- •3.3 Escape Depth of Secondary Electrons
- •3.8 Spatial Characteristics of Secondary Electrons
- •References
- •4: X-Rays
- •4.1 Overview
- •4.2 Characteristic X-Rays
- •4.2.1 Origin
- •4.2.2 Fluorescence Yield
- •4.2.3 X-Ray Families
- •4.2.4 X-Ray Nomenclature
- •4.2.6 Characteristic X-Ray Intensity
- •Isolated Atoms
- •X-Ray Production in Thin Foils
- •X-Ray Intensity Emitted from Thick, Solid Specimens
- •4.3 X-Ray Continuum (bremsstrahlung)
- •4.3.1 X-Ray Continuum Intensity
- •4.3.3 Range of X-ray Production
- •4.4 X-Ray Absorption
- •4.5 X-Ray Fluorescence
- •References
- •5.1 Electron Beam Parameters
- •5.2 Electron Optical Parameters
- •5.2.1 Beam Energy
- •Landing Energy
- •5.2.2 Beam Diameter
- •5.2.3 Beam Current
- •5.2.4 Beam Current Density
- •5.2.5 Beam Convergence Angle, α
- •5.2.6 Beam Solid Angle
- •5.2.7 Electron Optical Brightness, β
- •Brightness Equation
- •5.2.8 Focus
- •Astigmatism
- •5.3 SEM Imaging Modes
- •5.3.1 High Depth-of-Field Mode
- •5.3.2 High-Current Mode
- •5.3.3 Resolution Mode
- •5.3.4 Low-Voltage Mode
- •5.4 Electron Detectors
- •5.4.1 Important Properties of BSE and SE for Detector Design and Operation
- •Abundance
- •Angular Distribution
- •Kinetic Energy Response
- •5.4.2 Detector Characteristics
- •Angular Measures for Electron Detectors
- •Elevation (Take-Off) Angle, ψ, and Azimuthal Angle, ζ
- •Solid Angle, Ω
- •Energy Response
- •Bandwidth
- •5.4.3 Common Types of Electron Detectors
- •Backscattered Electrons
- •Passive Detectors
- •Scintillation Detectors
- •Semiconductor BSE Detectors
- •5.4.4 Secondary Electron Detectors
- •Everhart–Thornley Detector
- •Through-the-Lens (TTL) Electron Detectors
- •TTL SE Detector
- •TTL BSE Detector
- •Measuring the DQE: BSE Semiconductor Detector
- •References
- •6: Image Formation
- •6.1 Image Construction by Scanning Action
- •6.2 Magnification
- •6.3 Making Dimensional Measurements With the SEM: How Big Is That Feature?
- •Using a Calibrated Structure in ImageJ-Fiji
- •6.4 Image Defects
- •6.4.1 Projection Distortion (Foreshortening)
- •6.4.2 Image Defocusing (Blurring)
- •6.5 Making Measurements on Surfaces With Arbitrary Topography: Stereomicroscopy
- •6.5.1 Qualitative Stereomicroscopy
- •Fixed beam, Specimen Position Altered
- •Fixed Specimen, Beam Incidence Angle Changed
- •6.5.2 Quantitative Stereomicroscopy
- •Measuring a Simple Vertical Displacement
- •References
- •7: SEM Image Interpretation
- •7.1 Information in SEM Images
- •7.2.2 Calculating Atomic Number Contrast
- •Establishing a Robust Light-Optical Analogy
- •Getting It Wrong: Breaking the Light-Optical Analogy of the Everhart–Thornley (Positive Bias) Detector
- •Deconstructing the SEM/E–T Image of Topography
- •SUM Mode (A + B)
- •DIFFERENCE Mode (A−B)
- •References
- •References
- •9: Image Defects
- •9.1 Charging
- •9.1.1 What Is Specimen Charging?
- •9.1.3 Techniques to Control Charging Artifacts (High Vacuum Instruments)
- •Observing Uncoated Specimens
- •Coating an Insulating Specimen for Charge Dissipation
- •Choosing the Coating for Imaging Morphology
- •9.2 Radiation Damage
- •9.3 Contamination
- •References
- •10: High Resolution Imaging
- •10.2 Instrumentation Considerations
- •10.4.1 SE Range Effects Produce Bright Edges (Isolated Edges)
- •10.4.4 Too Much of a Good Thing: The Bright Edge Effect Hinders Locating the True Position of an Edge for Critical Dimension Metrology
- •10.5.1 Beam Energy Strategies
- •Low Beam Energy Strategy
- •High Beam Energy Strategy
- •Making More SE1: Apply a Thin High-δ Metal Coating
- •Making Fewer BSEs, SE2, and SE3 by Eliminating Bulk Scattering From the Substrate
- •10.6 Factors That Hinder Achieving High Resolution
- •10.6.2 Pathological Specimen Behavior
- •Contamination
- •Instabilities
- •References
- •11: Low Beam Energy SEM
- •11.3 Selecting the Beam Energy to Control the Spatial Sampling of Imaging Signals
- •11.3.1 Low Beam Energy for High Lateral Resolution SEM
- •11.3.2 Low Beam Energy for High Depth Resolution SEM
- •11.3.3 Extremely Low Beam Energy Imaging
- •References
- •12.1.1 Stable Electron Source Operation
- •12.1.2 Maintaining Beam Integrity
- •12.1.4 Minimizing Contamination
- •12.3.1 Control of Specimen Charging
- •12.5 VPSEM Image Resolution
- •References
- •13: ImageJ and Fiji
- •13.1 The ImageJ Universe
- •13.2 Fiji
- •13.3 Plugins
- •13.4 Where to Learn More
- •References
- •14: SEM Imaging Checklist
- •14.1.1 Conducting or Semiconducting Specimens
- •14.1.2 Insulating Specimens
- •14.2 Electron Signals Available
- •14.2.1 Beam Electron Range
- •14.2.2 Backscattered Electrons
- •14.2.3 Secondary Electrons
- •14.3 Selecting the Electron Detector
- •14.3.2 Backscattered Electron Detectors
- •14.3.3 “Through-the-Lens” Detectors
- •14.4 Selecting the Beam Energy for SEM Imaging
- •14.4.4 High Resolution SEM Imaging
- •Strategy 1
- •Strategy 2
- •14.5 Selecting the Beam Current
- •14.5.1 High Resolution Imaging
- •14.5.2 Low Contrast Features Require High Beam Current and/or Long Frame Time to Establish Visibility
- •14.6 Image Presentation
- •14.6.1 “Live” Display Adjustments
- •14.6.2 Post-Collection Processing
- •14.7 Image Interpretation
- •14.7.1 Observer’s Point of View
- •14.7.3 Contrast Encoding
- •14.8.1 VPSEM Advantages
- •14.8.2 VPSEM Disadvantages
- •15: SEM Case Studies
- •15.1 Case Study: How High Is That Feature Relative to Another?
- •15.2 Revealing Shallow Surface Relief
- •16.1.2 Minor Artifacts: The Si-Escape Peak
- •16.1.3 Minor Artifacts: Coincidence Peaks
- •16.1.4 Minor Artifacts: Si Absorption Edge and Si Internal Fluorescence Peak
- •16.2 “Best Practices” for Electron-Excited EDS Operation
- •16.2.1 Operation of the EDS System
- •Choosing the EDS Time Constant (Resolution and Throughput)
- •Choosing the Solid Angle of the EDS
- •Selecting a Beam Current for an Acceptable Level of System Dead-Time
- •16.3.1 Detector Geometry
- •16.3.2 Process Time
- •16.3.3 Optimal Working Distance
- •16.3.4 Detector Orientation
- •16.3.5 Count Rate Linearity
- •16.3.6 Energy Calibration Linearity
- •16.3.7 Other Items
- •16.3.8 Setting Up a Quality Control Program
- •Using the QC Tools Within DTSA-II
- •Creating a QC Project
- •Linearity of Output Count Rate with Live-Time Dose
- •Resolution and Peak Position Stability with Count Rate
- •Solid Angle for Low X-ray Flux
- •Maximizing Throughput at Moderate Resolution
- •References
- •17: DTSA-II EDS Software
- •17.1 Getting Started With NIST DTSA-II
- •17.1.1 Motivation
- •17.1.2 Platform
- •17.1.3 Overview
- •17.1.4 Design
- •Simulation
- •Quantification
- •Experiment Design
- •Modeled Detectors (. Fig. 17.1)
- •Window Type (. Fig. 17.2)
- •The Optimal Working Distance (. Figs. 17.3 and 17.4)
- •Elevation Angle
- •Sample-to-Detector Distance
- •Detector Area
- •Crystal Thickness
- •Number of Channels, Energy Scale, and Zero Offset
- •Resolution at Mn Kα (Approximate)
- •Azimuthal Angle
- •Gold Layer, Aluminum Layer, Nickel Layer
- •Dead Layer
- •Zero Strobe Discriminator (. Figs. 17.7 and 17.8)
- •Material Editor Dialog (. Figs. 17.9, 17.10, 17.11, 17.12, 17.13, and 17.14)
- •17.2.1 Introduction
- •17.2.2 Monte Carlo Simulation
- •17.2.4 Optional Tables
- •References
- •18: Qualitative Elemental Analysis by Energy Dispersive X-Ray Spectrometry
- •18.1 Quality Assurance Issues for Qualitative Analysis: EDS Calibration
- •18.2 Principles of Qualitative EDS Analysis
- •Exciting Characteristic X-Rays
- •Fluorescence Yield
- •X-ray Absorption
- •Si Escape Peak
- •Coincidence Peaks
- •18.3 Performing Manual Qualitative Analysis
- •Beam Energy
- •Choosing the EDS Resolution (Detector Time Constant)
- •Obtaining Adequate Counts
- •18.4.1 Employ the Available Software Tools
- •18.4.3 Lower Photon Energy Region
- •18.4.5 Checking Your Work
- •18.5 A Worked Example of Manual Peak Identification
- •References
- •19.1 What Is a k-ratio?
- •19.3 Sets of k-ratios
- •19.5 The Analytical Total
- •19.6 Normalization
- •19.7.1 Oxygen by Assumed Stoichiometry
- •19.7.3 Element by Difference
- •19.8 Ways of Reporting Composition
- •19.8.1 Mass Fraction
- •19.8.2 Atomic Fraction
- •19.8.3 Stoichiometry
- •19.8.4 Oxide Fractions
- •Example Calculations
- •19.9 The Accuracy of Quantitative Electron-Excited X-ray Microanalysis
- •19.9.1 Standards-Based k-ratio Protocol
- •19.9.2 “Standardless Analysis”
- •19.10 Appendix
- •19.10.1 The Need for Matrix Corrections To Achieve Quantitative Analysis
- •19.10.2 The Physical Origin of Matrix Effects
- •19.10.3 ZAF Factors in Microanalysis
- •X-ray Generation With Depth, φ(ρz)
- •X-ray Absorption Effect, A
- •X-ray Fluorescence, F
- •References
- •20.2 Instrumentation Requirements
- •20.2.1 Choosing the EDS Parameters
- •EDS Spectrum Channel Energy Width and Spectrum Energy Span
- •EDS Time Constant (Resolution and Throughput)
- •EDS Calibration
- •EDS Solid Angle
- •20.2.2 Choosing the Beam Energy, E0
- •20.2.3 Measuring the Beam Current
- •20.2.4 Choosing the Beam Current
- •Optimizing Analysis Strategy
- •20.3.4 Ba-Ti Interference in BaTiSi3O9
- •20.4 The Need for an Iterative Qualitative and Quantitative Analysis Strategy
- •20.4.2 Analysis of a Stainless Steel
- •20.5 Is the Specimen Homogeneous?
- •20.6 Beam-Sensitive Specimens
- •20.6.1 Alkali Element Migration
- •20.6.2 Materials Subject to Mass Loss During Electron Bombardment—the Marshall-Hall Method
- •Thin Section Analysis
- •Bulk Biological and Organic Specimens
- •References
- •21: Trace Analysis by SEM/EDS
- •21.1 Limits of Detection for SEM/EDS Microanalysis
- •21.2.1 Estimating CDL from a Trace or Minor Constituent from Measuring a Known Standard
- •21.2.2 Estimating CDL After Determination of a Minor or Trace Constituent with Severe Peak Interference from a Major Constituent
- •21.3 Measurements of Trace Constituents by Electron-Excited Energy Dispersive X-ray Spectrometry
- •The Inevitable Physics of Remote Excitation Within the Specimen: Secondary Fluorescence Beyond the Electron Interaction Volume
- •Simulation of Long-Range Secondary X-ray Fluorescence
- •NIST DTSA II Simulation: Vertical Interface Between Two Regions of Different Composition in a Flat Bulk Target
- •NIST DTSA II Simulation: Cubic Particle Embedded in a Bulk Matrix
- •21.5 Summary
- •References
- •22.1.2 Low Beam Energy Analysis Range
- •22.2 Advantage of Low Beam Energy X-Ray Microanalysis
- •22.2.1 Improved Spatial Resolution
- •22.3 Challenges and Limitations of Low Beam Energy X-Ray Microanalysis
- •22.3.1 Reduced Access to Elements
- •22.3.3 At Low Beam Energy, Almost Everything Is Found To Be Layered
- •Analysis of Surface Contamination
- •References
- •23: Analysis of Specimens with Special Geometry: Irregular Bulk Objects and Particles
- •23.2.1 No Chemical Etching
- •23.3 Consequences of Attempting Analysis of Bulk Materials With Rough Surfaces
- •23.4.1 The Raw Analytical Total
- •23.4.2 The Shape of the EDS Spectrum
- •23.5 Best Practices for Analysis of Rough Bulk Samples
- •23.6 Particle Analysis
- •Particle Sample Preparation: Bulk Substrate
- •The Importance of Beam Placement
- •Overscanning
- •“Particle Mass Effect”
- •“Particle Absorption Effect”
- •The Analytical Total Reveals the Impact of Particle Effects
- •Does Overscanning Help?
- •23.6.6 Peak-to-Background (P/B) Method
- •Specimen Geometry Severely Affects the k-ratio, but Not the P/B
- •Using the P/B Correspondence
- •23.7 Summary
- •References
- •24: Compositional Mapping
- •24.2 X-Ray Spectrum Imaging
- •24.2.1 Utilizing XSI Datacubes
- •24.2.2 Derived Spectra
- •SUM Spectrum
- •MAXIMUM PIXEL Spectrum
- •24.3 Quantitative Compositional Mapping
- •24.4 Strategy for XSI Elemental Mapping Data Collection
- •24.4.1 Choosing the EDS Dead-Time
- •24.4.2 Choosing the Pixel Density
- •24.4.3 Choosing the Pixel Dwell Time
- •“Flash Mapping”
- •High Count Mapping
- •References
- •25.1 Gas Scattering Effects in the VPSEM
- •25.1.1 Why Doesn’t the EDS Collimator Exclude the Remote Skirt X-Rays?
- •25.2 What Can Be Done To Minimize gas Scattering in VPSEM?
- •25.2.2 Favorable Sample Characteristics
- •Particle Analysis
- •25.2.3 Unfavorable Sample Characteristics
- •References
- •26.1 Instrumentation
- •26.1.2 EDS Detector
- •26.1.3 Probe Current Measurement Device
- •Direct Measurement: Using a Faraday Cup and Picoammeter
- •A Faraday Cup
- •Electrically Isolated Stage
- •Indirect Measurement: Using a Calibration Spectrum
- •26.1.4 Conductive Coating
- •26.2 Sample Preparation
- •26.2.1 Standard Materials
- •26.2.2 Peak Reference Materials
- •26.3 Initial Set-Up
- •26.3.1 Calibrating the EDS Detector
- •Selecting a Pulse Process Time Constant
- •Energy Calibration
- •Quality Control
- •Sample Orientation
- •Detector Position
- •Probe Current
- •26.4 Collecting Data
- •26.4.1 Exploratory Spectrum
- •26.4.2 Experiment Optimization
- •26.4.3 Selecting Standards
- •26.4.4 Reference Spectra
- •26.4.5 Collecting Standards
- •26.4.6 Collecting Peak-Fitting References
- •26.5 Data Analysis
- •26.5.2 Quantification
- •26.6 Quality Check
- •Reference
- •27.2 Case Study: Aluminum Wire Failures in Residential Wiring
- •References
- •28: Cathodoluminescence
- •28.1 Origin
- •28.2 Measuring Cathodoluminescence
- •28.3 Applications of CL
- •28.3.1 Geology
- •Carbonado Diamond
- •Ancient Impact Zircons
- •28.3.2 Materials Science
- •Semiconductors
- •Lead-Acid Battery Plate Reactions
- •28.3.3 Organic Compounds
- •References
- •29.1.1 Single Crystals
- •29.1.2 Polycrystalline Materials
- •29.1.3 Conditions for Detecting Electron Channeling Contrast
- •Specimen Preparation
- •Instrument Conditions
- •29.2.1 Origin of EBSD Patterns
- •29.2.2 Cameras for EBSD Pattern Detection
- •29.2.3 EBSD Spatial Resolution
- •29.2.5 Steps in Typical EBSD Measurements
- •Sample Preparation for EBSD
- •Align Sample in the SEM
- •Check for EBSD Patterns
- •Adjust SEM and Select EBSD Map Parameters
- •Run the Automated Map
- •29.2.6 Display of the Acquired Data
- •29.2.7 Other Map Components
- •29.2.10 Application Example
- •Application of EBSD To Understand Meteorite Formation
- •29.2.11 Summary
- •Specimen Considerations
- •EBSD Detector
- •Selection of Candidate Crystallographic Phases
- •Microscope Operating Conditions and Pattern Optimization
- •Selection of EBSD Acquisition Parameters
- •Collect the Orientation Map
- •References
- •30.1 Introduction
- •30.2 Ion–Solid Interactions
- •30.3 Focused Ion Beam Systems
- •30.5 Preparation of Samples for SEM
- •30.5.1 Cross-Section Preparation
- •30.5.2 FIB Sample Preparation for 3D Techniques and Imaging
- •30.6 Summary
- •References
- •31: Ion Beam Microscopy
- •31.1 What Is So Useful About Ions?
- •31.2 Generating Ion Beams
- •31.3 Signal Generation in the HIM
- •31.5 Patterning with Ion Beams
- •31.7 Chemical Microanalysis with Ion Beams
- •References
- •Appendix
- •A Database of Electron–Solid Interactions
- •A Database of Electron–Solid Interactions
- •Introduction
- •Backscattered Electrons
- •Secondary Yields
- •Stopping Powers
- •X-ray Ionization Cross Sections
- •Conclusions
- •References
- •Index
- •Reference List
- •Index
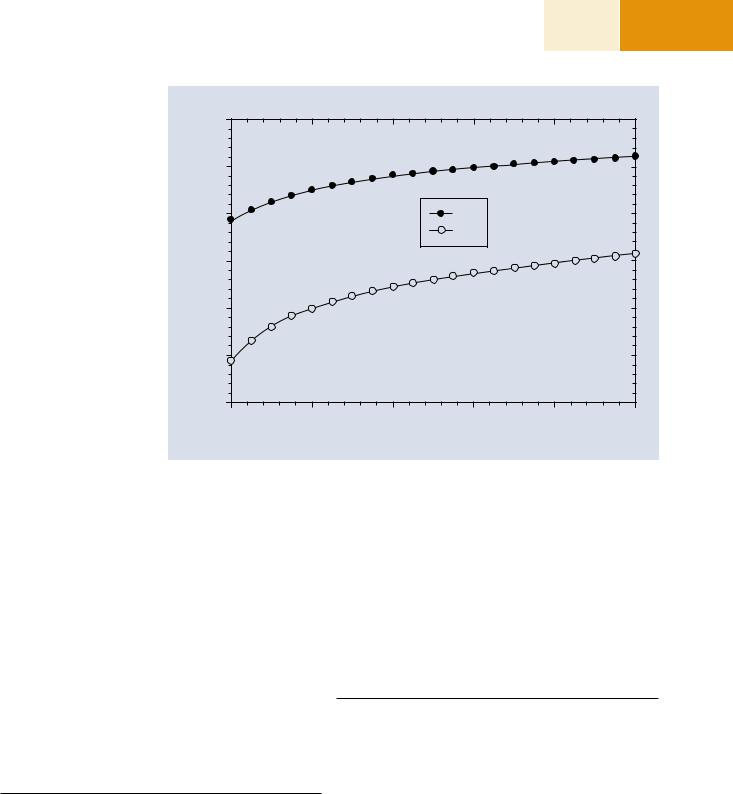
25.2 · What Can Be Done To Minimize gas Scattering in VPSEM? |
|
|
|
451 |
25 |
|
|
|
|
|
|
||
. Fig. 25.12 Comparison of the |
|
|
|
|
|
|
scattering skirt for He and O2 |
1.0 |
|
VPSEM 100 Pa 10 mm GPL |
|
|
|
|
|
|
|
|
|
|
|
0.9 |
|
|
|
|
|
intensity |
0.8 |
|
|
He |
|
|
|
|
|
O2 |
|
|
|
electron |
0.7 |
|
|
|
|
|
|
|
|
|
|
|
|
Cumulative |
0.6 |
|
|
|
|
|
|
|
|
|
|
|
|
|
0.5 |
|
|
|
|
|
|
0.4 |
|
|
|
|
|
|
0 |
10 |
20 |
30 |
40 |
50 |
|
|
|
Radial distance from beam center (micrometers) |
|
4.T, the sample chamber temperature (K): The scattering skirt is reduced by operating at the lowest possible temperature.
5.L, the gas path length (m). The shorter the gas path length, the smaller the gas scattering skirt, as shown in
. Fig. 25.4, where the skirt is compared for gas path lengths of 3 mm, 5 mm, and 10 mm. Note that the gas path length appears in Eq. (25.1) with a 3/2 power, so that the skirt radius is more sensitive to this parameter than the other parameters in Eq. (25.1). A modification to the vacuum system of the VPSEM that minimizes the gas path length consists of using a small diameter tube to extend the high vacuum of the electron column into the sample chamber.
25.2.1\ Workarounds ToSolve Practical
Problems
Gas scattering effects can be minimized but not avoided, and for many VPSEM measurements and in situ experiments, the microscopist/microanalyst may be significantly constrained in the extent to which any of the parameters in
Eq. (25.1) can actually be changed to reduce the gas scattering skirt without losing the advantages of VPSEM operation. The measured EDS spectrum is always compromised, but by carefully choosing the problems to study, successful X-ray analysis can still be performed. A useful way to consider the impact of gas scattering is the general concentration level at which the remote scattering corrupts the measured spectrum:
Major constituent: concentration C > 0.1 mass fraction Minor constituent: 0.01 ≤C ≤0.1
Trace constituent: C < 0.01
Depending on the exact nature of the specimen, gas scattering will almost always introduce spectral artifacts at the trace and minor constituent levels, and in severe cases artifacts will appear at the level of apparent major constituents.
25.2.2\ Favorable Sample Characteristics
Given that the LVSEM operating conditions have been selected to minimize the gas scattering skirt, what specimen types are most likely to yield useful microanalysis results? If most of the gas scattering skirt falls on background material that contains an element or elements that are different from the elements of the target and of no interest, then by following a measurement protocol to identify the extraneous elements, the measured spectrum can still have value for identifying the elements within the target area, always recognizing that the target is being excited by the focused beam and the skirt.
Particle Analysis
Particle samples comprise a broad class of problems related to the environment, technology, forensics, failure analysis, and other areas. Particles are very often insulating in nature so that a conductive coating is required for examination in the conventional high vacuum SEM, and the complex morphologies
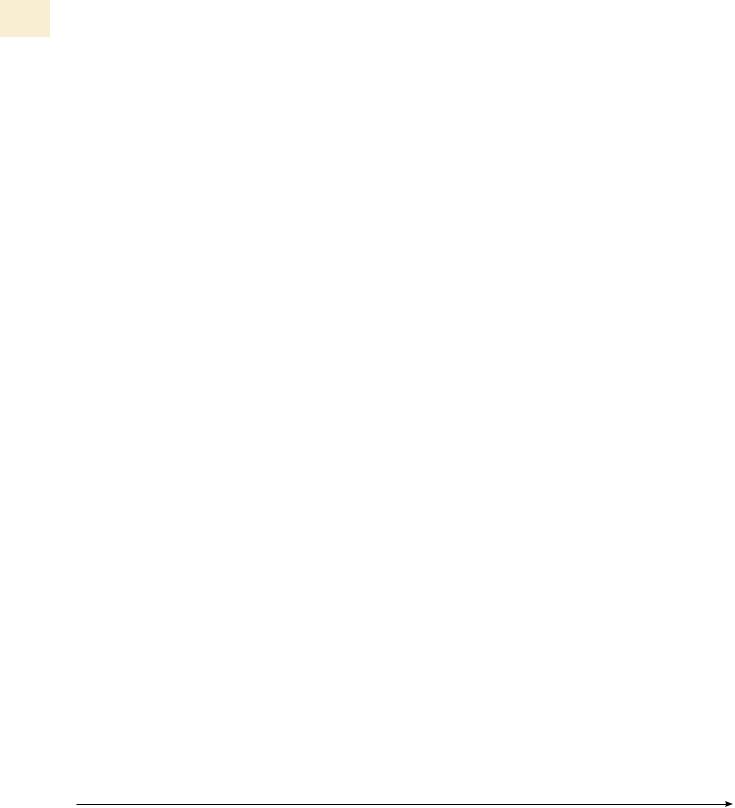
\452 Chapter 25 · Attempting Electron-Excited X-Ray Microanalysis in the Variable Pressure Scanning Electron Microscope (VPSEM)
of particles often make it difficult to apply a suitable coating. 25 The VPSEM with its charge dissipation through gas ionization is an attractive alternative to achieve successful particle imaging. When VPSEM X-ray analysis measurements are needed to characterize particles, specimen preparation is critical to achieve a useful result. There are many methods available for particle preparation, but the general goal for successful VPSEM X-ray analysis is to broadly disperse the particles on a suitable substrate so that the unscattered beam and the innermost intense portion of the skirt immediately surrounding the beam can be placed on individual particles without exciting nearby particles. The more distant portions of the beam skirt may still excite other particles in the dispersion, but the relative fraction of the electrons that falls on these particles is likely to be sufficiently small that the artifacts introduced in the measured spectrum will be equivalent to trace (C <0.01
mass fraction) constituents.
\1.\ When particles are collected on a smooth (i.e., not tortuous path) medium such as a porous polycarbonate filter, the loaded filter can be studied directly in the VPSEM with no preparation other than to attach a portion of the filter to a support stub. Prior to attempting X-ray measurements of individual particles, the X-ray spectrum of the filter material should be measured under the VPSEM operating conditions as the first stage of determining the analytical blank (that is, the spectral contributions of all the materials involved in the preparation except the specimen itself). In addition to revealing the elemental constituents of the filter, this blank spectrum will also reveal the contribution of the environmental gas to the spectrum.
\2.\ When particles are to be transferred from the collection medium, such as a tortuous path filter, or simply obtained from a loose mass in a container, the choice of the sample substrate is the first question to resolve. Conceding that VPSEM operation will lead to significant remote scattering that will excite the substrate, the sample substrate should be chosen to consist of an element that is not of interest in the analysis of individual particles. Carbon
is a typical choice for the substrate material, but if the analysis of carbon in the particles is important, then an alternative material such as beryllium (but beware of the health hazards of beryllium and its oxide) or boron can be selected. If certain higher atomic number elements can be safely ignored in the analysis, then additional materials may be suitable for substrates, such as aluminum, silicon,
germanium, or gold (often as a thick film evaporated on silicon). Again, whatever the choice of substrate, the X-ray spectrum of the bare substrate should be measured to establish the analytical blank prior to analyzing particles on that substrate.
. Figure 25.13a shows a VPSEM image of a particle cluster prepared on carbon tape (which has a blank spectrum consisting of major C and minor O from the polymer base) and the EDS X-ray spectrum obtained with the beam placed on particle “A”. The spectrum is seen to contain a high intensity peak for Si, lower intensity peaks for O, Al, and K, and peaks just at the threshold of detection for Ca, Ti, and Fe. With the other particles nearby, how much of this spectrum can be reliably assigned to particle “A”? A local “operational blank” can be measured by placing the beam on several nearby substrate locations so that focused beam only excites the substrate while the skirt continues to excite the specimen over its extended reach. Examples of the “working blanks” for this particular specimen are shown in . Fig. 25.13b, c and are revealed to be surprisingly similar, considering the separation of location “BL3” from “BL1” and the particle array. Inspection of these working blanks show Al and Si at the minor level, and Ca and Fe at the trace level, as estimated from the peak-to-background ratio. Thus, the interpretation of the spectrum of particle “A” can make use of the local analytical blank, as shown in . Fig. 25.13d. The major Al and Si peaks are not significantly perturbed by the low blank contributions for these elements, and the minor K is not present in the working blank and therefore it can be considered valid. However, the low levels of Ca and Fe observed in the blank are similar to those in the spectrum on particle “A”, and thus they should be removed these from consideration as legitimate trace constituents. Note that despite the size of particle “A,” which is approximately 50 μm in its longest dimension, it must be remembered that measurement sensitivity to any possible heterogeneity within particle “A” is likely to be lost in the VPSEM mode because of the large fraction of the incident current that is transferred within the 25-μm radius, as demonstrated in . Table 25.1. Another example of the use of the working blank is shown in . Fig. 25.13e for particle “D”. Here the Ca is much higher than in the working blank, and so it can be considered a legitimate particle constituent, as can the Mg, which is not in the working blank. The low Fe peak is similar in both the particle spectrum and the working blank, so it must not be considered legitimate.
. Fig. 25.13 a VPSEM image of a cluster of particles and an EDS X-ray spectrum measured with the beam placed on one of them, particle “A.” b EDS X-ray spectrum measured with the beam placed on the substrate at location BL1 so that only the beam skirt excites the particles. c EDS X-ray spectrum measured with the beam placed on the substrate at
location BL3 so that only the beam skirt excites the particles. d Use of analytical blank to aid interpretation of the EDS spectrum of Particle “A.” e Use of analytical blank to aid interpretation of the EDS spectrum of Particle “A”
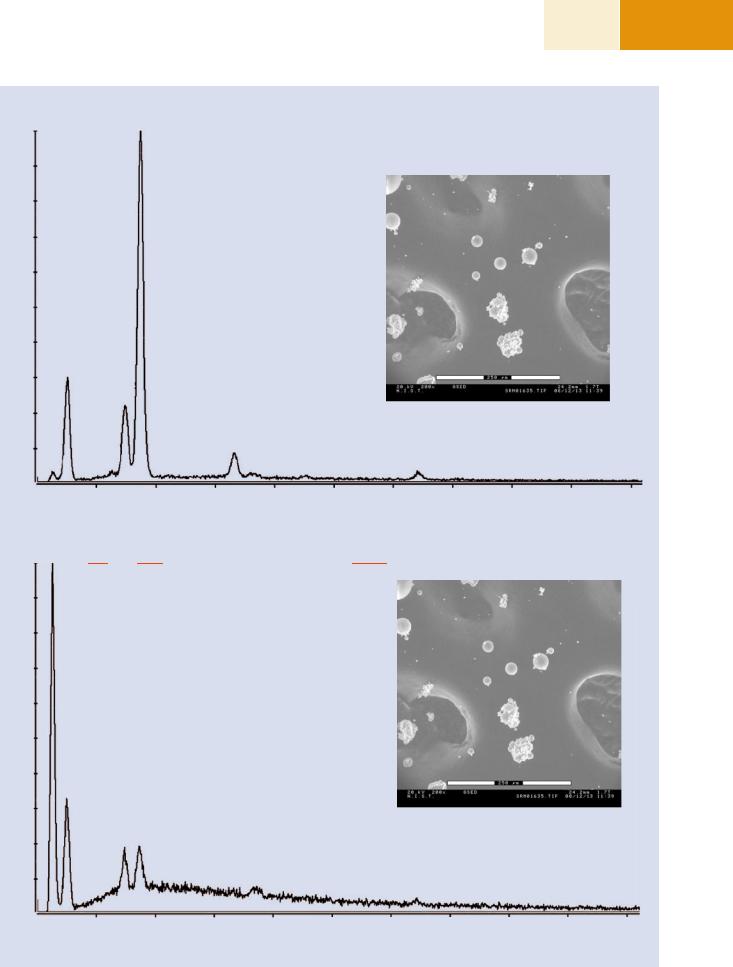
453 |
25 |
25.2 · What Can Be Done To Minimize gas Scattering in VPSEM?
a |
|
|
|
|
|
|
|
|
|
|
|
|
|
|
|
|
|
|
|
|
|
|
|
|
|
3453 |
Ch#: |
|
|
Ch kV; |
|
|
|
|
Work: 51 |
Results: 0 |
Marker: |
|
|
|
|
|
|
|
|
|
|
|
|||
261 |
2.6100 |
Fe |
26 |
|
|
|
|
|
|
||||||||||||||||
|
|
|
|
|
|
|
|
|
SiKa |
|
|
|
|
|
|
|
|
|
|
|
|
|
|
||
|
|
|
|
|
|
|
|
|
|
|
|
|
Particle “A” |
|
|
|
|
|
|
|
BL3 |
|
|||
|
|
|
|
|
|
|
|
|
|
|
|
|
|
|
|
|
|
|
|
E |
|
|
|
|
|
|
|
|
|
|
|
|
|
|
|
|
|
|
|
|
|
|
|
|
|
BL2 |
B |
C |
|
|
|
|
|
|
|
|
|
|
|
|
|
|
|
|
|
|
|
|
|
|
|
|
|
|
|
|
|
|
|
|
|
|
|
|
|
|
|
|
|
|
|
|
|
|
|
|
|
D |
|
BL1 |
|
|
|
|
|
|
|
|
|
|
|
|
|
|
|
|
|
|
|
|
|
|
|
|
|
|
|
|
|
|
|
|
|
|
|
|
|
|
|
|
|
|
|
|
|
|
|
|
|
|
|
|
A |
|
|
|
O Ka |
|
|
|
Al Ka |
|
|
|
|
|
|
|
|
|
|
|
|
|
|
||||||
|
|
|
|
|
|
|
|
|
|
|
|
|
|
|
|
|
|
|
|||||||
|
C Ka |
|
MgKa |
|
|
|
|
|
K Ka |
Ti Ka |
|
|
FeKa |
|
|
|
|
|
|
||||||
|
|
|
|
|
|
|
|
S |
Ka |
CaKa |
|
|
FeKb1 |
|
|
|
|
|
|||||||
|
|
|
|
|
|
|
|
|
|
|
|
|
Ti Kb1 |
|
|
|
|
|
|
|
|
||||
0.00 |
1.00 |
|
2.00 |
3.00 |
4.00 |
5.00 |
|
|
6.00 |
7.00 |
|
|
8.00 |
9.00 |
10.00 |
||||||||||
|
|
|
|
|
|
|
|
|
|
|
|
|
|
Photon energy (keV) |
|
|
|
|
|
|
|||||
b |
|
Ch# |
|
|
|
|
|
|
|
|
|
|
|
|
|
|
|
|
|
|
|
|
|
|
|
1396 |
|
|
ChkV: |
|
|
Work: 101 |
Results: 0 |
Marker: |
|
|
|
|
|
|
|
|
|
||||||||
: 274 |
2.7400 |
Fe |
26 |
|
|
|
|
|
|
|
|||||||||||||||
|
C |
k |
|
|
|
|
|
|
|
|
|
|
|
|
|
|
|
|
|
|
|
||||
|
|
|
|
|
|
|
|
|
|
|
|
|
|
|
|
|
|
|
|
|
|
|
|
BL3 |
|
|
|
|
|
|
|
|
|
|
|
|
|
|
|
|
|
|
|
|
|
|
E |
|
|
|
|
|
|
|
|
|
|
|
|
|
|
|
|
|
|
|
|
|
|
|
|
|
B L2 |
B |
C |
|
|
|
|
|
|
|
|
|
|
|
|
|
|
|
|
|
|
|
|
|
|
|
|
|
|
|
D BL
Operational blank at “BL
A
|
O |
K |
|
|
|
|
|
|
|
|
|
|
|
Si K |
|
|
|
|
|
|
|
|
|
|
|
|
|
|
|
|
|
|
|
|
|
|
|
|
Al K |
|
|
|
|
|
|
|
|
|
|
|
|
|
CaK |
|
FeK |
|
|
|
|
|
|
|
|
|
|
|
|
|
|
|
|
0.00 |
|
1.00 |
2.00 |
3.00 |
4.00 |
5.00 |
6.00 |
7.00 |
8.00 |
9.00 |
10.00 |
|
|
|
|
|
|
Photon energy (keV) |
|
|
|
|
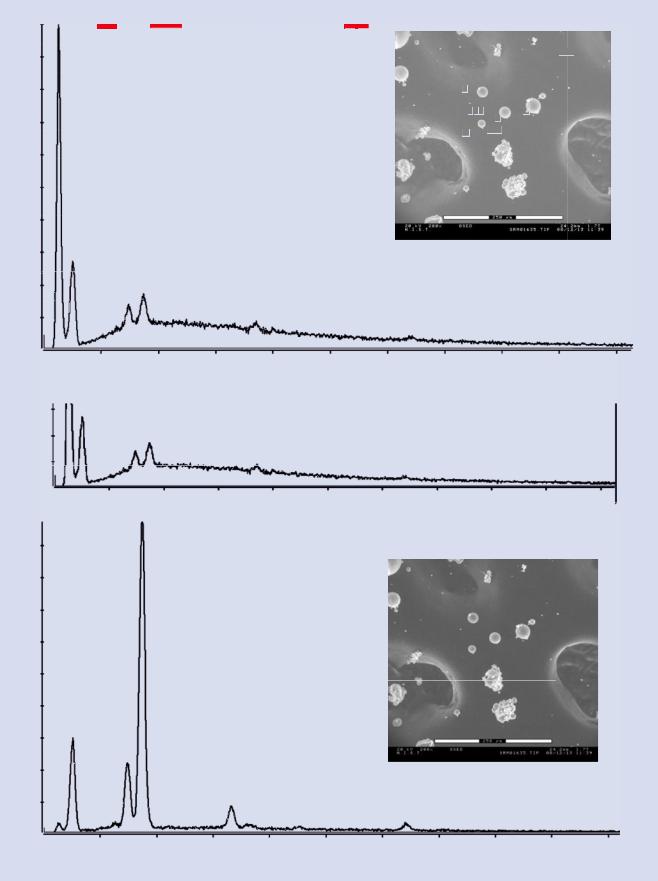
\454 Chapter 25 · Attempting Electron-Excited X-Ray Microanalysis in the Variable Pressure Scanning Electron Microscope (VPSEM)
25 |
c 3718 |
Ch#: |
|
Ch kV: |
|
Work: 269 |
Results: 0 |
Marke:r: |
|
|
|
|
2.6600 |
|
|||||||||
266 |
Fe 26 |
|
|||||||||
|
C |
K |
|
|
|
|
BL3 |
||||
|
|
|
|
|
|||||||
|
|
|
|
|
|
|
|
|
|
|
|
|
|
|
|
|
Operational blank at “BL3 |
|
|
E |
|
||
|
|
|
|
|
|
|
|
|
|
B L2 |
C |
|
|
|
|
|
|
|
|
|
|
|
B |
|
|
|
|
|
|
|
|
|
|
D |
BL1 |
A
|
O K |
|
|
|
|
|
|
|
|
|
|
|
|
SiK |
|
|
|
|
|
|
|
|
|
|
Al K |
|
|
|
|
|
|
|
|
|
|
|
|
|
|
CaK |
|
|
|
|
|
|
|
|
|
|
|
|
|
FeK |
|
|
|
|
|
0.00 |
1.00 |
2.00 |
3.00 |
4.00 |
5.00 |
6.00 |
7.00 |
8.00 |
9.00 |
10.00 |
|
|
|
|
|
|
Photon energy (keV) |
|
|
|
|
|
|
d |
|
|
|
|
|
|
|
|
|
|
|
|
|
SiKa |
|
|
Operational blank adjacent to A |
|
|
|
|||
|
AlKa |
|
|
|
|
|
|||||
|
|
|
|
CaKa |
|
FeKa |
|
|
|
|
|
|
|
|
|
|
|
|
|
|
|
|
|
30.00 |
1.00 |
2.00 |
3.00 |
4.00 |
5.00 |
6.00 |
7.00 |
8.00 |
|
9.00 |
10.00 |
|
|
|
|
|
Photon energy (keV) |
|
|
|
|
|
|
|
|
SiKa |
|
|
|
|
|
|
|
|
|
|
|
|
|
Particle “A” |
|
|
|
|
BL3 |
|
|
|
|
|
|
|
|
|
E |
|
|
|
|
|
|
|
|
|
|
|
BL2 |
B |
C |
|
|
|
|
|
|
|
|
|
D |
BL1 |
|
|
|
|
|
|
|
|
|
|
|
A |
|
|
|
0 |
Ka |
|
|
|
|
|
|
|
|
|
|
|
ALKa |
|
|
|
|
|
|
|
|
|
|
|
|
|
K |
Ka |
|
FeKa |
|
|
|
|
|
C |
MgKa |
S |
Ka |
|
TiKa |
|
|
|
|
|
|
Ka |
|
|
CaKa |
TiKb1 |
|
FeKb1 |
|
|
|
|
|
0.00 |
1.00 |
2.00 |
3.00 |
4.00 |
5.00 |
6.00 |
7.00 |
8.00 |
|
9.00 |
10.00 |
|
|
|
|
|
Photon energy (keV) |
|
|
|
|
|
. Fig. 25.13 (continued)
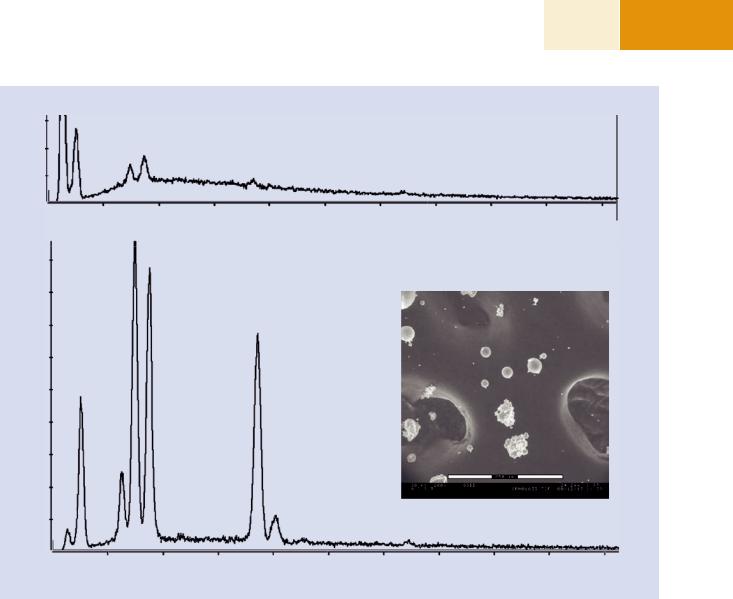
455 |
25 |
25.2 · What Can Be Done To Minimize gas Scattering in VPSEM?
e |
|
|
|
|
|
|
|
|
|
|
|
|
SiKa |
|
|
|
|
Operational blank adjacent to D |
|
||
|
|
AlKa |
|
CaKa |
|
|
|
|
|
|
|
|
|
|
|
|
FeKa |
|
|
|
|
|
|
|
|
|
|
|
|
|
|
|
0.00 |
1.00 |
2.00 |
3.00 |
4.00 |
5.00 |
6.00 |
7.00 |
8.00 |
9.00 |
10.00 |
|
|
|
|
|
Photon energy (keV) |
|
|
|
Particle “D”
BL3
CaKa
E
O |
Ka |
|
|
|
|
|
|
|
|
BL2 |
B |
C |
|
|
|
|
|
|
|
|
|
D |
BL1 |
|
|
||
|
|
|
|
|
|
|
|
|
|
|
|
A |
|
|
MgKa |
|
|
|
|
|
|
|
|
|
|
|
|
C K |
a |
S K |
a |
K |
K |
a |
TiK |
a |
FeKa |
|
|
|
|
|
|
|
|
|
|
|
|
|
|
||||
|
|
|
|
|
|
|
|
TiKb1 |
|
FeKb1 |
|
|
|
0.00 |
1.00 |
2.00 |
|
3.00 |
|
|
4.00 |
5.00 |
6.00 |
7.00 |
8.00 |
9.00 |
10.00 |
|
|
|
|
|
|
|
|
Photon energy (keV) |
|
|
|
|
. Fig. 25.13 (continued)