
ЛЕКЦИИ ФШФС_2007 / НАШИ СТАТЬИ / JCTE667_Ильичев_ РЭ 6_2005 Англ
.pdf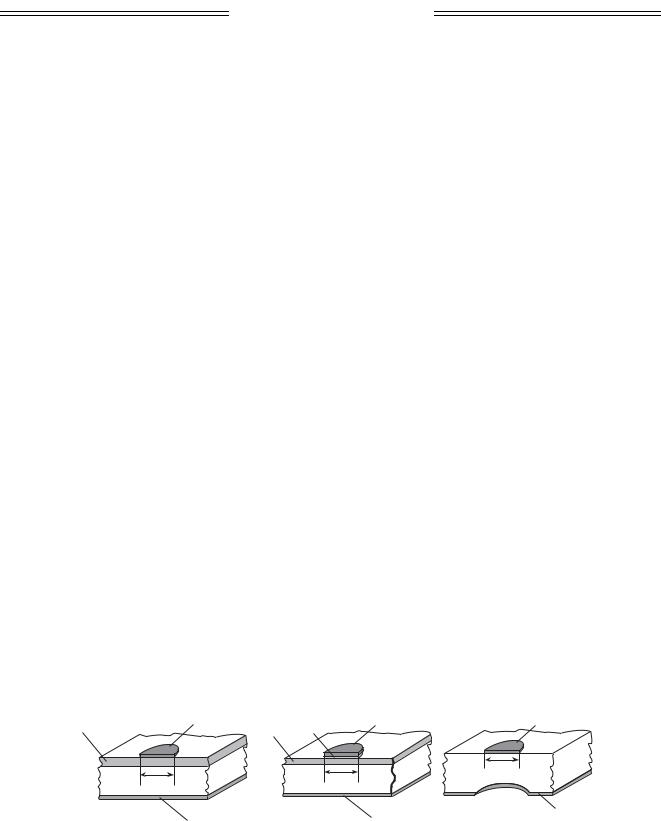
Journal of Communications Technology and Electronics, Vol. 50, No. 6, 2005, pp. 667–672. Translated from Radiotekhnika i Elektronika, Vol. 50, No. 6, 2005, pp. 729–735. Original Russian Text Copyright © 2005 by Zhigal’skii, Bespalov, Gorbatsevich, Il’ichev, Shmelev, Andrushchenko, Rodin.
English Translation Copyright © 2005 by MAIK “Nauka /Interperiodica” (Russia).
PHYSICAL PROCESSES
IN ELECTRON DEVICES
Effect of Deep Trapping Levels on the Excess Noise in GaAs High-Energy Particle Detectors
G. P. Zhigal’skii, V. A. Bespalov, A. A. Gorbatsevich, E. A. Il’ichev,
S. S. Shmelev, S. A. Andrushchenko, and M. S. Rodin
Received October 29, 2004
Abstract—Results are presented from experimental studies of deep levels and the excess noise in active layers of various types of GaAs detector structures developed for the detection of high-energy particles and X-ray and γ radiation. A correlation is revealed between the level of the excess noise (1/f γ) and parameters of deep energy centers of receiving layers of GaAs ionizing-radiation detectors and their spectrometric characteristics.
INTRODUCTION
Estimates of the microparticle and γ-quantum detection efficiencies of solid-state detectors manufactured from non-silicon materials often show that GaAs detectors offer the greatest promise [1]. In particular, such detectors can be successfully used in solution of positioning problems, for radiometry and spectrometry of charged-particle and γ-quantum beams in manufacturing areas characterized by increased temperature and radiation backgrounds, and for medical diagnostics. In [1] these estimates were obtained with allowance for the following: cross sections of particle (quantum) exchange processes; the efficiency of the subsequent readout of the current of nonequilibrium carriers into an external circuit; and manufacturability, radiation hardness, and thermal stability of the analyzed materials. Parameters determining the choice of the detector material were as follows: the ordinal number of an element and the specific energy loss of the particle or the quantum, the ratio of the carrier lifetime in bands of nonlocalized states to the time required to transfer the carriers into an external circuit, the threshold energy of formation of nonequilibrium electron–hole pairs, the threshold energy of the defect-formation process, and dark currents.
An important problem for the developers of i-GaAs detectors is the high level of electric noise that limits the detector sensitivity, especially the level of the excess noise, including the generation–recombination (GR) noise and the 1/f γ (flicker) noise [2].
Since the level of background impurities in GaAs exceeds that in single-crystal Si and semi-insulating GaAs is a compensated semiconductor, we can a priori expect a high level of excess noise in GaAs detectors, which limits their sensitivity.
This paper is devoted to the experimental investigation of the relation between spectrometric characteristics of GaAs detectors and the excess noise (a combination of the recombination noise and the 1/f γ noise).
1. SPECIMENS
AND INVESTIGATION TECHNIQUES
Investigations were performed on three groups of test detector structures having the vertical designs schematically shown in Fig. 1.
The first group comprised the Schottky barrier structures Me–n–-GaAs–n+-G‡Äs–Me (see Fig. 1a, where V–Au is the Schottky contact, n-GaAs is the epitaxial layer, n+-GaAs is the substrate, and AuGeNi is the ohmic contact [3]).
(a) |
|
(b) |
|
(c) |
V–Au |
|
V–Au |
|
V–Au |
n-GaAs |
n-GaAs |
p+-GaAs |
|
|
|
|
|
|
|
Dn+-GaAs |
|
D n+-GaAs |
D |
i-GaAs |
|
|
|||
|
|
|
||
AuGeNi |
|
AuGeNi |
|
AuGeNi |
|
|
|
Fig. 1. Schematics of the types of detectors studied: (a) Schottky barrier test structures (Me−n–-GaAs–n+-G‡Äs–Me), (b) structures with a p–n junction (Me–p+-GaAs–n–-GaAs–n+-GaAs–Me), and (c) resistive structures (Me–i-GaAs–Me).
667
668 |
ZHIGAL’SKII et al. |
The second group comprised structures with a p–n junction (Fig. 1b). Here, p+-GaAs is the epitaxial layer with the p-type conduction, n-GaAs is the epitaxial layer with the n-type conduction and n+-GaAs is the substrate.
The third group of specimens comprised Me–i- GaAs–Me structures (Fig. 1c) manufactured as follows: a V–Au double-layered contact was formed on the right side of the wafer and the contact made of the AuGe alloy was formed on the back side of the wafer. Over the contact, a layer of Ni was deposited [3].
Active (acceptor) layers of Schottky barrier and p−n-junction epitaxial structures were manufactured from n- and p-type GaAs layers grown using the chloride method of gaseous epitaxy [3].
Active (acceptor) regions of detectors belonging to the third group were manufactured using commercially produced wafers of semi-insulating GaAs (i-GaAs) compensated by its own defects. Detector structures were manufactured using “membrane” technology. The central part of the crystal with a diameter D ≈ 5 mm and linear dimensions of 6 × 8 mm2 was thinned from the back side of the substrate to a thickness of ~100 µm. Then, an AuGeNi ohmic contact was formed on the substrate and a V–Au contact with D ≈ 4 mm was formed in the middle part of the wafer’s right side.
As is known [4] the operating principle of all described detector cells is based on measuring (in the reverse-bias mode) the current of nonequilibrium charge carriers created by the flux of elementary particles or γ radiation. Therefore, in this paper electrophysical characteristics of detector structures and the excess noise that were both measured in the reverse-bias mode are presented.
The excess noise was measured using the technique described in [5]. For this purpose the structure being tested was fed a bias voltage through a 10-MΩ resistor. The noise of the detector structure was amplified by a low-noise preamplifier connected on 2P303A fieldeffect transistors fed from galvanic elements. The amplified signal entered a spectrum analyzer. The level of the preamplifier’s intrinsic noise at a frequency of 20 Hz was no more than 10–14 V2 /Hz under no-load conditions and no more than 10–17 V2 /Hz under shortcircuit conditions, which was substantially less than that of the noise level in the structures under study. The power spectral density (PSD) of the structure noise was measured in the frequency range 20–60 kHz in the presence of bias voltages up to –100 V at the barrier structure.
For each detector specimen, electrophysical characteristics determining the kinetics of the transfer of current carriers were measured. By measuring characteristics of the Hall effect in a semi-insulating (pure) GaAs layer, typical values of specific resistance ρv, mobility µ, and equilibrium carrier concentration Nn were obtained for different specimens. The respective values
of these parameters were the following: ρv ≈ 107– 108 Ω cm, µ ≈ (4–5) × 103 cm2 /V s, and Nn ≈ 5 × 106−108 cm–3.
Current–voltage characteristics were used to determine leakage (dark) currents for reverse-bias voltages of up to –100 V at temperatures T ranging from 296 to 400 K.
C–V-metry was used to determine the dependence of depletion depth d of active regions on the reverse-bias voltage.
The detectors’ radiation hardness was studied for the action of high-energy electrons with energy E = 4 MeV and a radiation dose of ≈107 rad, as well as the electromagnetic radiation from a 60Co source with energy E = 1.25 MeV and a radiation dose of about 3 × 107 rad. Deep levels (DLs) in the detector’s target material were studied with the help of a contactless nondestructive method of deep-level relaxation optoelectronic spectroscopy (DLROS) [6]. In this study, energies Et (in eV) of the DL trap centers, capture cross sections St (in cm2), and product fNt (in cm–3) of filling function f and trap concentration Nt were determined.
The DLROS method [6] consists the contactless recording in both the electron–hole and trap systems of relaxation processes that occur under periodic excitation of these systems by light and a quasi-equilibrium variation in the specimen temperature. This method allows measurement of the relaxation time of both the electron–hole and trap systems in semiconducting and semi-insulating materials and determination of the deep levels’ type, energy characteristics, and concentration. For specimens with trap concentration Nt less than equilibrium carrier concentration Nn , this method allows estimation of the value of parameter Nn and determination of the type of the material conduction.
The nondestructive character of the diagnostics used by this method is a consequence of the process where the heated specimen is placed between plates of a capacitor and the controlled region is irradiated through one of the capacitor’s semitransparent plates by the electromagnetic radiation with both a quantum energy equal to or higher than the energy gap width and a periodically varying amplitude. The local character of these diagnostics in the wafer plane and along the wafer depth is determined by the dimensions of the light probe and the effective diffusion distance, respectively.
The physical phenomena behind this method are related to GR processes in the irradiated region of the specimen and to formation of the diffusion–drift potential. The light incident onto the specimen penetrates to depth α–1, where α is the light absorption coefficient. In this case the type of carrier dominating in the probed volume is that with the longest lifetime in allowed bands. This observation follows from the validity of the stationary equation p/τp = n/τn [7], where p and n are concentrations of holes and electrons and τp and τn are
JOURNAL OF COMMUNICATIONS TECHNOLOGY AND ELECTRONICS Vol. 50 No. 6 2005
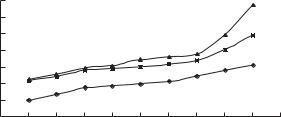
EFFECT OF DEEP TRAPPING LEVELS ON THE EXCESS NOISE |
669 |
lifetimes of holes and electrons, respectively. During both the diffusion and the drift in the field created by the potential difference of the near-surface contact, nonequilibrium carriers move into the depths of the specimen, which substantially changes the pattern of the equilibrium population of deep centers and creates a volume charge in this region. This, in turn, changes the near-surface potential distribution, and the periodic variation in the irradiation intensity of the probed region of the specimen causes, accordingly, a periodic variation in the photopotential, which permits application of capacitance recording methods. In the case of a pulsed excitation, which is sometimes more convenient in practice, the value of the photopotential changes from maximum level ϕm to some level ϕ0 reached at the time corresponding to the end of the pause between the light pulses.
2. EXPERIMENTAL RESULTS
S × 1014, V2 /Hz
107 |
|
|
|
|
3 |
|
|
|
|
|
|
105 |
|
|
|
|
2 |
|
|
|
|
|
|
|
|
|
|
|
1 |
103 |
|
|
|
|
|
101 |
25 |
45 |
65 |
85 |
105 |
5 |
– U, V
Fig. 2. Dependence of the noise PSD in detector structures on the reverse-bias voltage at a frequency of 20 Hz. Curves 1–3 correspond, respectively, to the Schottky barrier structure
(Me–n–-GaAs–n+-G‡Äs–Me) with the receiving layer formed without special doping, the structure with a p–n
junction (Me–p+-GaAs–n–-GaAs–n+-GaAs–Me), and the
Schottky barrier structure (Me–n–-GaAs–n+-G‡Äs–Me) with a Si-doped receiving layer.
Let us consider the results of comparative experimental investigations of high-energy particle detectors with compensated and pure epitaxial GaAs layers.
Electrophysical parameters of active layers of four specimens of the studied detector structures are listed in Table 1, where numbers I and III denote test structures with the Schottky barrier of the first group (Fig. 1a), number II denotes the test structure with the p–n junction (Fig. 1b), and number IV denotes the structure with bulk active resistive layer Me–i-GaAs–Me (Fig. 1c). Here, d is the thickness of a pure layer; µ is the electron mobility in a pure layer; Et (eV), St (cm2), and fNt (cm–3) are initial values of the trap-center energy, the capture cross section, and the product of the filling function and the trap concentration in a pure GaAs layer, respectively; and D is the deep energy center of the donor type. Note that wafers of detector structures I and III (Table 1) were cut from a monocrystalline GaAs ingot grown using the Czochralski method [3]. The active layer of structure III was doped with Si during the growth, whereas no special doping was applied to receiving layers of structure I.
For the trap spectra in active layers, the following results were obtained: for detector structures I and II,
trap-center energy Et ≈ 0.23 eV (donor) and trap concentration in the pure layer Nt ≈ 1013 cm–3 and, for detector structure III, Et ≈ 0.6 eV and Nt ≈ 5 × 1013 cm–3. Both donor and acceptor DLs were found in the studied specimens, but donor levels dominated in GR processes.
As seen from Table 1, structures of type III have a higher concentration of equilibrium current carriers and a higher density of trap levels. In structures of this type, trap levels with energy Et = 0.6 eV appeared.
Analyzing the experimental results on study of the excess noise as a function of applied voltage U, we can select two regions of operation of barrier detectors:
(1) the optimum operating mode corresponding to U ≈ 20–30 V and (2) the prebreakdown operating mode characterized by an increased level of the excess noise (Fig. 2) corresponding to U ≈ 70–80 V.
Figure 3 shows frequency dependences of the PSD of the excess noise in specimens I–IV for reverse-bias voltages listed in Table 2.
In the frequency range 20 Hz to 1 kHz, spectrum shape index γ ≈ 1.83–1.99 for specimens I, II, and III. For specimen IV with a high DL concentration (fNt ≈
Table 1. Electrophysical parameters of detector structures
|
|
Electrophysical parameters of the structure layers |
|
|||||||
|
|
|
|
|
|
|
|
|
|
|
Types of detector structures |
Nn, cm–3 |
d, µm |
µ, cm2/(V s) |
|
|
DL parameters |
|
|||
|
|
|
|
|
|
|
|
|||
|
E |
, eV |
|
S |
, cm2 |
|
fN , cm–3 |
|||
|
|
|
|
|
|
|||||
|
|
|
|
t |
|
|
t |
|
|
t |
I: Me–n–-GaAs–n+-GaAs |
1013 |
29 |
4400 |
0.23 D |
|
2 × 10–17 |
|
1013 |
||
II: Me–p+-GaAs–n–-GaAs–n+-GaAs |
1013 |
29 |
4400 |
0.23 D |
|
2 × 10–17 |
|
1013 |
||
III: Me–n–-GaAs–n+-GaAs |
3 × 1014 |
30 |
3900 |
0.6 D |
|
5 × 10–13 |
|
5 × 1013 |
||
IV: Me–i–-GaAs–Me |
7 × 106 |
≈150 |
4300 |
0.48 D |
|
2 × 10–14 |
|
7 × 1014 |
JOURNAL OF COMMUNICATIONS TECHNOLOGY AND ELECTRONICS Vol. 50 No. 6 2005
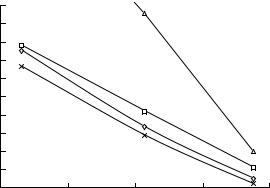
670 |
|
|
|
ZHIGAL’SKII et al. |
|
S × 1018, V2/Hz |
|
|
|
|
olution of a detector, R; and integral energy noise SI in |
1010 |
|
|
|
|
the studied specimens are listed in Table 2. Parameters |
|
|
|
|
|
R and SI were used to select and analyze characteristics |
108 |
|
|
|
|
of the detector operating in the accumulation mode. |
|
|
|
|
For spectrometric investigations, sources of α parti- |
|
2 |
|
|
4 |
|
|
106 |
|
3 |
|
cles with energy E = 5.147 and 5.499 MeV were used |
|
|
|
|
for specimen I and sources with E = 5.499 and |
||
1 |
|
|
|
|
|
|
|
|
|
5.456 MeV were used for specimen II. |
|
104 |
|
|
|
|
In the optimum reverse-bias operating mode of bar- |
|
|
|
|
|
rier detector cells, dark leakage currents IL in devices |
102 |
|
|
|
|
with an active region area of ≈0.12 cm2 were as follows: |
|
|
|
|
|
(1) IL ≈ 140 nA for targets made of semi-insulating |
100 |
|
|
|
|
materials, (2) IL ≈ 10 nA for targets made of pure GaAs |
101 |
102 |
103 |
104 |
105 |
with the background electron concentration Nn ≈ 3 × |
|
|
|
|
f, Hz |
1013 cm–3 and containing barrier metal–semiconductor |
Fig. 3. Frequency dependences of the PSD of the 1/f γ noise |
contacts, (3) IL ≈ 6.5 nA for targets made of low-doped |
||||
GaAs with electron concentration Nn ≈ 3 × 1014 cm–3 |
|||||
at the reverse bias for different types of detector structures. |
and containing barrier metal–semiconductor contacts, |
||||
Curves 1–4 correspond, respectively, to the Schottky barrier |
|||||
structure (Me–n–-GaAs–n+-G‡Äs–Me) with the receiving |
and (4) IL ≈ 45 nA for targets made of pure i-GaAs with |
||||
layer formed without special doping and a reverse bias of |
a p–n junction. |
Ub = –22 V, the structure with a p–n junction (Me–p+-
GaAs–n–-GaAs–n+-GaAs–Me) and Ub = –30 V, the Schot-
tky barrier structure (Me–n–-GaAs–n+-G‡Äs–Me) with the Si-doped receiving layer and Ub = –30 V, and the resistive
structure (Me–i-GaAs–Me) with Ub = –40 V.
A correlation between the level of the excess noise in the dark operating mode and trap concentration fNt was revealed for most detector structures. It was also found that the leakage current is not always an informative parameter for estimating the energy resolution of the detector and the threshold sensitivity of the detector.
7 × 1014 cm–3), γ ≈ 4.63. The noise component with a 1/f 3 spectrum was observed in i-GaAs Schottky barrier structures [5, 8, 9]. However, the mechanism lying behind the formation of such spectra is not yet completely understood. As seen from Fig. 3, Schottky barrier detector structures manufactured on pure GaAs substrates (type I) have the lowest noise level and detector structures of type IV have the highest noise level. An increased noise level in structure II as compared to structure I apparently arises because epitaxial layers p+ and n– are grown in different manufacturing cycles (see Fig. 1b).
Experimental values of the noise DSP at frequencies of 20 and 1330 Hz; leakage currents of depleting electrode IL , where Vd is the depletion voltage; energy res-
A most informative parameter for determining the quality of charged-particle detectors is the level of excess noise [5, 9, 10]. Therefore, the method involving measurements of excess noise by test structures can be recommended for the nondestructive testing of GaAs based devices and integrated microassemblies at both the manufacturing and finished-product stages.
In comparative spectrometric investigations performed at different temperatures on Si and GaAs detectors, it has been found that both Si detectors and detectors formed on semi-insulating GaAs demonstrate a substantial charge loss of nonequilibrium carriers at temperatures above 50°C. In addition, the energy resolution of these detectors (especially, Si detectors) rapidly lowers and the level of the equivalent energy noise increases. Since this phenomenon is not observed in
Table 2. Threshold and operating characteristics of detectors
|
|
|
|
Electrophysical parameters of detectors |
|
|
|||
Type of detector |
|
|
|
|
|
|
|
||
PSD, V2/Hz |
|
|
|
optimum operating mode |
|
||||
structure |
|
|
|
|
|
|
|
|
|
|
20 Hz |
1330 Hz |
|
Vd, V |
IL, nA |
|
R, keV |
SI , keV |
|
|
|
|
|
|
|
|
|
|
|
I |
1.09 × 10–13 |
1.58 |
× 10–15 |
|
–22 |
<15 |
|
35 |
18.7 |
II |
4.35 × 10–11 |
2.94 |
× 10–15 |
|
–30 |
45 |
|
15.1 |
8.3 |
III |
6.26 × 10–11 |
1.6 |
× 10–14 |
|
–30 |
6–7 |
|
141 |
9.1 |
IV |
>10–8 |
3.36 × 10–9 |
|
–40 |
140 |
|
40 |
– |
JOURNAL OF COMMUNICATIONS TECHNOLOGY AND ELECTRONICS Vol. 50 No. 6 2005
EFFECT OF DEEP TRAPPING LEVELS ON THE EXCESS NOISE |
|
|
671 |
|||||||
Table 3. Influence of radiation on basic device parameters |
|
|
|
|
|
|
|
|||
|
|
|
|
|
|
|
|
|
|
|
|
|
|
|
|
Type of irradiation |
|
|
|
|
|
|
|
|
|
|
|
|
|
|||
Type of the structure |
HEE (4 MeV and 107 rad) |
γ radiation from 60Co (1.25 |
MeV and 3 × 107 rad) |
|||||||
|
|
|
|
|
|
|
|
|
|
|
|
∆I/I |
∆V/V |
|
∆S/S |
∆I/I |
∆V/V |
∆S/S |
|
∆K/K |
∆τ/τ |
|
|
|
|
|
|
|
|
|
|
|
Uniformly doped epitaxial layers |
–0.7 |
0.4 |
|
–0.6 |
+0.25 |
+0.15 |
–0.10 |
– |
–0.3 |
|
δ—1012/3 |
–0.4 |
0.25 |
|
–0.10 |
– |
– |
– |
|
– |
– |
δ—3 × 1012/1 |
–0.3 |
0.18 |
|
–0.07 |
+0.18 |
+0.10 |
~0.02 |
<0.02 |
<0.02 |
GaAs detectors made on pure layers, it seems reasonable to associate it with GR processes involving DLs. Apparently, these processes are responsible for a high level of excess noise in GaAs detectors formed on compensated materials (1–2 orders of magnitude higher than the noise PSD in detectors formed on pure layers). For detectors formed on a narrow-band silicon material, a substantial deterioration of the detector parameters is caused by an increased rate of the band-to-band thermal generation and recombination.
Because of a substantial electromagnetic background existing in manufacturing areas of reactors and accelerators, it is necessary to install electronic units in the immediate vicinity of detectors. Therefore, it is not possible to exclude the negative influence of ionizing radiations on devices of the service units.
It is known that the radiation hardness of GaAs devices and circuits is an order of magnitude larger than the radiation hardness of similar Si circuits [4].
In this paper we present the results of comparative measurements of the effect of high-energy particles and γ quanta on substrates and epitaxial structures (ESs) with pure GaAs layers, as well as GaAs devices manufactured on structures with different architectures and doping levels. The need for investigations of semi-insulating substrates and pure layers is related to the possible use of such structures as active regions of detector cells. Tests of GaAs devices and circuits for radiation hardness are required because, in some cases, signal preprocessing devices should be installed in the immediate vicinity of a detector (in the active zone of a reactor or an accelerator).
The results on the influence of radiation on the source GaAs material used for manufacturing ionizing-radia- tion detectors and on basic parameters of the components (transistors and amplifiers) of GaAs integrated circuits (ICs) used for the preprocessing of signals from ioniz- ing-radiation detectors are presented in Tables 3 and 4.
The types of ionizing radiations used were highenergy electrons (HEEs) with an energy of ~4 MeV and γ quanta with an energy of ~1.25 MeV. The source materials for GaAs detectors were semi-insulating GaAs with a specific resistance ρ ≈ 5 × 107 Ω cm, a concentration of deep energy centers of the donor type Nt ≈ 1015 cm–3, and the deep center energies Et ≈ 0.87 and 0.38 eV, as well as pure epitaxial GaAs layers that were
manufactured using the method of gaseous epitaxy and had a background concentration of doping and deep impurities of ~5 × 1013 cm–3. A contactless nondestructive method [6] was used to control the type, energy spectrum, and concentration of deep energy centers in the source material and in the material irradiated using the aforementioned doses of ionizing radiations. During the irradiation of the IC components, relative variations in the saturation current (∆I/I), the threshold voltage (∆V/V), and the transconductance (∆S/S) of Schottky barrier field-effect transistors (SFETs), as well as in gains (∆K/K) of GaAs amplifiers based on Schmidt triggers, were controlled. Variations in the photopotential relaxation time (∆τ/τ) were also controlled. The transistors studied were manufactured on epitaxial GaAs structures. The active transistor layers were either layers with a uniform doping profile (N ~ 1017 cm–3 and d ~ 0.25 µm) or layers with a complex doping profile: single-layered and three-layered δ-doped structures with electron concentrations of 3 × 1012 and 1012 cm–2 in each δ layer, respectively, which are denoted in Tables 3 and by δ—3 × 1012/1 and δ—1012/3.
It has been found that, when semi-insulating GaAs layers are exposed to the action of an electron beam with a radiation dose of ~107 rad and an energy of ~4 MeV, the energy position of a localized deep donor center slightly shifts to the shortwave region. This shift is accompanied by the appearance of a new donor center with an energy of ~0.38 eV and a concentration of ~1014 cm–3. Here, changes in the total concentration of localized centers are small and, almost coinciding with concentrations recorded in the substrates before irradiation (~7 × 1014 cm–3), only slightly affect energy parameters of deep energy centers in a semi-insulating substrate. In the case of pure epitaxial layers with a background concentration of the deep impurity of ~8 × 1013 cm–3 and an energy of ~0.6 eV, we observed a substantial change in the concentration of localized centers owing to the appearance of a donor center with an energy of ~0.35 eV at concentrations of ~2 × 1014 cm–3. If we take into account that the levels of doped active layers of transistors operating in the normally open channel mode are ~1017 cm–3, it is difficult to expect substantial changes in the output and control characteristics of transistors and circuits. However, substantial changes
JOURNAL OF COMMUNICATIONS TECHNOLOGY AND ELECTRONICS Vol. 50 No. 6 2005
672 |
|
ZHIGAL’SKII et al. |
|
|
|
|
|||
Table 4. Influence of radiation on electrophysical properties of semi-insulating GaAs and pure epitaxial layers |
|
||||||||
|
|
|
|
|
|
|
|
|
|
|
|
|
|
State |
|
|
|
||
|
|
|
|
|
|
|
|
|
|
Type of structure |
before HEE irradiation |
after HEE irradiation |
before γ-quantum |
after γ-quantum |
|||||
irradiation |
irradiation |
||||||||
|
|
|
|
|
|||||
|
|
|
|
|
|
|
|
|
|
|
Nt , cm–3 |
Et , eV |
Nt , cm–3 |
Et , eV |
Nt , cm–3 |
Et , eV |
Nt , cm–3 |
Et , eV |
|
|
|
|
|
|
|
|
|
|
|
Pure n–-GaAs epitaxial layer |
8 × 1013 |
0.6 |
2 × 1014 |
0.35 |
8 × 1013 |
0.6 |
1014 |
0.20 |
|
Semi-insulating GaAs |
7 × 1014 |
0.82 |
1015 |
0.87, 0.38 |
7 × 1014 |
0.82 |
7 × 1014 |
0.82 |
were observed in the saturation current (∆I/I), threshold voltages (∆V/V), and transconductance (∆S/S), especially for transistors with uniformly doped active layers (see Table 3), and can be associated with the bulk character of damage created by radiation fluxes. Hence, it seems expedient (see experimental data in Table 4) to use structures containing δ-doped layers as components of the structure.
In the process of exposing tested specimens to γ quanta with a radiation dose of ~3 × 107 rad, saturation currents increase and the transconductance decreases; however, the most substantial changes are also observed in the characteristics of transistors formed on δ-doped structures. No changes were noticed in the trap energy spectra of semi-insulating substrates, and in pure bulk GaAs layers, formation of a smaller donor center with a concentration slightly exceeding the initial level was recorded (see Table 4). Seemingly, it is the formation of a rather small donor center that causes the growth of the saturation current by increasing the threshold voltage and decreasing the slope. A decrease in the photopotential’s characteristic relaxation time was also observed (δτ/τ).
Investigations of the influence of high-energy electrons (with E = 4 MeV and a dose of ≈107 rad) and γ radiation from a 60Co source (with E = 1.25 MeV and a dose of ~3 × 107 rad) have shown that structures formed on pure epitaxial layers (types I and II), which have the highest energy resolution characteristics, are most vulnerable to radiation-induced damage. The semi-insulat- ing i-GaAs layer (type IV) was found to be the most tolerable to irradiation. However, our investigations have shown that detectors manufactured on this material have a high noise level and a lowered energy resolution.
CONCLUSIONS
The results of complex experimental investigations allow us to consider GaAs as a promising material for high-energy particle detectors. Offering almost the same sensitivity and the energy resolution as Si detectors, GaAs detectors allow a substantial extension of the range of operating temperatures and lowering of the operating voltage to 20–30 V, which is important for the control of radiation detectors and for the signal processing performed by peripheral circuits.
Detector structures formed on pure layers (types I and II), which have the highest spectral sensitivity, demonstrate the lowest tolerance to radiation-induced damages. The GaAs semi-insulator (type IV) is the most irradiation-tolerant material. However, these detector cells have a high level of excess noise and a low energy resolution. For detector structures, a correlation between the level of excess noise in the dark mode and the trap concentration was revealed.
The results presented on the investigation into the influence of ionizing-radiation and high-energy particle fluxes on characteristics of GaAs detector cells facilitate correct selection of the architecture of working structures and of the design of devices developed for long-time, stable operation in areas with an increased temperature and radiation backgrounds.
REFERENCES
1.V. A. Bespalov, A. V. Vorontsov, A. A. Gorbatsevich, et al., Zh. Tekh. Fiz. 74 (3), 28 (2004) [Tech. Phys. 49, 310 (2004)].
2.M. Buckingham, Noise in Electron Devices and Systems
(Ellis Horwood, Chichester, UK, 1983; Mir, Moscow, 1986).
3.M. Shur, GaAs Devices and Circuits (Plenum, New York, 1987; Mir, Moscow, 1991).
4.E. R. Astvatsatur’yan, D. V. Gromov, and V. M. Lomako,
Radiation Effects in GaAs Devices and Integrated Circuits
(Universitetskoe, Minsk, 1992) [in Russian].
5.G. P. Zhigal’skii, A. A. Gorbatsevich, V. V. Lyublin, et al., Radiotekh. Elektron. (Moscow) 47, 244 (2002) [J. Commun. Technol. Electron. 47, 223 (2002)].
6.E. A. Il’ichev, Zh. Tekh. Fiz. 68 (5), 141 (1998).
7.S. M. Ryvkin, Photometric Phenomena in Semiconductors (Fizmatlit, Moscow, 1963) [in Russian].
8.R. L. Bates, S. D. Auria, S. J. Gowdy, et al., in Proc. 4th Workshop on GaAs Detectors and Related Compounds, San Milano, Italy, March 19–21, 1995 (World Sci., 1996), p. 1.
9.G. P. Zhigal’skii, A. A. Gorbatsevich, V. V. Lublin, et al., in Noise in Physical Systems and 1/f Fluctuation (Proc. 15th Int. Conf., Ginesville, Fla., USA, 2001) (World Sci., 2001), p. 253.
10.G. P. Zhigal’skii, Usp. Fiz. Nauk 173, 465 (2003) [Phys. Usp. 46, 449 (2003)].
JOURNAL OF COMMUNICATIONS TECHNOLOGY AND ELECTRONICS Vol. 50 No. 6 2005