
Биоинженерия / ТИ_печень(органы_ЖКТ) / Bioengineering_Liver_Transplantation
.pdfBioengineering 2019, 6, 95
17.Powers, M.J.; Janigian, D.M.; Wack, K.E.; Baker, C.S.; Stolz, D.B.; Gri th, L.G. Functional Behavior of Primary Rat Liver Cells in a Three-Dimensional Perfused Microarray Bioreactor. Tissue Eng. 2002, 8, 499–513. [CrossRef] [PubMed]
18.Allen, J.W.; Bhatia, S.N. Improving the next generation of bioartificial liver devices. Semin. Cell Dev. Biol. 2002, 13, 447–454. [CrossRef] [PubMed]
19.Bao, J.; Fisher, J.; Nyberg, S.L. Liver Regeneration and Tissue Engineering. In Tissue Engineering in Regenerative Medicine; Humana Press: New York, NY, USA, 2011; pp. 315–332.
20.Melchels, F.P.W.; Domingos, M.A.N.; Klein, T.J.; Malda, J.; Bartolo, P.J.; Hutmacher, D.W. Additive Manufacturing of Tissues and Organs. Prog. Polym. Sci. 2012, 37, 1079–1104. [CrossRef]
21.Jose, R.R.; Rodriguez, M.J.; Dixon, T.A.; Omenetto, F.G.; Kaplan, D.L. Evolution of Bioinks and Additive Manufacturing Technologies for 3D Bioprinting. ACS Biomater. Sci. Eng. 2016, 2, 1662–1678. [CrossRef]
22.Gross, B.C.; Erkal, J.L.; Lockwood, S.Y.; Chen, C.; Spence, D.M. Evaluation of 3D Printing and Its Potential Impact on Biotechnology and the Chemical Sciences. Anal. Chem. 2014, 86, 3240–3253. [CrossRef] [PubMed]
23.Choi, J.Y.; Das, S.; Theodore, N.D. Advances in 2D/3D Printing of Functional Nanomaterials and Their Applications. ECS J. Solid State Sci. Technol. 2015, 4, 3001–3009. [CrossRef]
24.Wang, X.; Yan, Y.; Zhang, R. Rapid prototyping as a tool for manufacturing bioartificial livers. Trends Biotechnol. 2007, 25, 505–513. [CrossRef] [PubMed]
25.Derby, B. Printing and prototyping of tissues and sca olds. Science 2012, 338, 921–926. [CrossRef] [PubMed]
26.Choi, Y.J.; Yi, H.G.; Kim, S.W.; Cho, D.W. 3D Cell Printed Tissue Analogues: A New Platform for Theranostics. Theranostics 2017, 7, 3118–3137. [CrossRef] [PubMed]
27.Iwami, K.; Noda, T.; Ishida, K.; Morishima, K.; Nakamura, M.; Umeda, N. Bio rapid prototyping by extruding/aspirating/refilling thermoreversible hydrogel. Biofabrication 2010, 2, 014108. [CrossRef] [PubMed]
28.Xu, T.; Zhao, W.; Zhu, J.M.; Albanna, M.Z.; Yoo, J.J.; Atala, A. Complex heterogeneous tissue constructs containing multiple cell types prepared by inkjet printing technology. Biomaterials 2013, 34, 130–139. [CrossRef]
29.Guillemot, F.; Souquet, A.; Catros, S.; Guillotin, B.; Lopez, J.; Faucon, M.; Pippenger, B.; Bareille, R.; Remy, M.; Bellance, S.; et al. High-throughput laser printing of cells and biomaterials for tissue engineering. Acta Biomater. 2010, 6, 2494–2500. [CrossRef]
30.Guillotin, B.; Souquet, A.; Catros, S.; Duocastella, M.; Pippenger, B.; Bellance, S.; Bareille, R.; Remy, M.; Bordenave, L.; Amedee, J.; et al. Laser assisted bioprinting of engineered tissue with high cell density and microscale organization. Biomaterials 2010, 31, 7250–7256. [CrossRef]
31.Bohandy, J.; Kim, B.F.; Adrian, F.J. Metal deposition from a supported metal film using an excimer laser. J. Appl. Phys. 1986, 60, 1538–1539. [CrossRef]
32.Guillemot, F.; Souquet, A.; Catros, S.; Guillotin, B. Laser-assisted cell printing: Principle, physical parameters versus cell fate and perspectives in tissue engineering. Nanomedicine 2010, 5, 507–515. [CrossRef] [PubMed]
33.Zhang, X.; Zhang, Y. Tissue Engineering Applications of Three-Dimensional Bioprinting. Cell Biophys. 2015, 72, 777–782. [CrossRef] [PubMed]
34.Karaiskou, A.; Zergioti, I.; Fotakis, C.; Kapsetaki, M.; Kafetzopoulos, D. Microfabrication of biomaterials by the sub-ps laser-induced forward transfer process. Appl. Surf. Sci. 2003, 208, 245–249. [CrossRef]
35.Othon, C.M.; Wu, X.; Anders, J.J.; Ringeisen, B.R. Single-cell printing to form three-dimensional lines of olfactory ensheathing cells. Biomed. Mater. 2008, 3, 034101. [CrossRef] [PubMed]
36.Gruene, M.; Deiwick, A.; Koch, L.; Schlie, S.; Unger, C.; Hofmann, N.; Bernemann, I.; Glasmacher, B.; Chichkov, B. Laser Printing of Stem Cells for Biofabrication of Sca old-Free Autologous Grafts. Tissue Eng. Part C Methods 2011, 17, 79–87. [CrossRef] [PubMed]
37.Koch, L.; Deiwick, A.; Schlie, S.; Michael, S.; Gruene, M.; Coger, V.; Zychlinski, D.; Schambach, A.; Reimers, K.; Vogt, P.M.; et al. Skin tissue generation by laser cell printing. Biotechnol. Bioeng. 2012, 109, 1855–1863. [CrossRef] [PubMed]
38.Koch, L.; Kuhn, S.; Sorg, H.; Gruene, M.; Schlie, S.; Gaebel, R.; Polchow, B.; Reimers, K.; Stoelting, S.; Ma, N.; et al. Laser Printing of Skin Cells and Human Stem Cells. Tissue Eng. Part C Methods 2010, 16, 847–854. [CrossRef] [PubMed]
39.Catros, S.; Guillotin, B.; Bacakova, M.; Fricain, J.C.; Guillemot, F. E ect of laser energy, substrate film thickness and bioink viscosity on viability of endothelial cells printed by Laser-Assisted Bioprinting. Appl. Surf. Sci. 2011, 257, 5142–5147. [CrossRef]
100
Bioengineering 2019, 6, 95
40.Keriquel, V.; Guillemot, F.; Arnault, I.; Guillotin, B.; Miraux, S.; Amedee, J.; Fricain, J.C.; Catros, S. In vivobioprinting for computerand robotic-assisted medical intervention: Preliminary study in mice. Biofabrication 2010, 2, 014101. [CrossRef] [PubMed]
41.Gudapati, H.; Dey, M.; Ozbolat, I. A comprehensive review on droplet-based bioprinting: Past, present and future. Biomaterials 2016, 102, 20–42. [CrossRef]
42.Hansen, C.J.; Saksena, R.; Kolesky, D.B.; Vericella, J.J.; Kranz, S.J.; Muldowney, G.P.; Christensen, K.T.; Lewis, J.A. High-Throughput Printing via Microvascular Multinozzle Arrays. Adv. Mater. 2013, 25, 96–102. [CrossRef] [PubMed]
43.Kim, J.D.; Choi, J.S.; Kim, B.S.; Choi, Y.C.; Cho, Y.W. Piezoelectric inkjet printing of polymers: Stem cell patterning on polymer substrates. Polymer 2010, 51, 2147–2154. [CrossRef]
44.Xu, T.; Jin, J.; Gregory, C.; Hickman, J.J.; Boland, T. Inkjet printing of viable mammalian cells. Biomaterials 2005, 26, 93–99. [CrossRef] [PubMed]
45.Skardal, A.; Zhang, J.; Prestwich, G.D. Bioprinting vessel-like constructs using hyaluronan hydrogels crosslinked with tetrahedral polyethylene glycol tetracrylates. Biomaterials 2010, 31, 6173–6181. [CrossRef] [PubMed]
46.Phillippi, J.A.; Miller, E.; Weiss, L.; Huard, J.; Waggoner, A.; Campbell, P. Microenvironments Engineered by Inkjet Bioprinting Spatially Direct Adult Stem Cells Toward Muscleand Bone-Like Subpopulations. Stem Cells 2008, 26, 127–134. [CrossRef] [PubMed]
47.Skardal, A.; Mack, D.; Kapetanovic, E.; Atala, A.; Jackson, J.D.; Yoo, J.; Soker, S. Bioprinted Amniotic Fluid-Derived Stem Cells Accelerate Healing of Large Skin Wounds. Stem Cells Transl. Med. 2012, 1, 792–802. [CrossRef] [PubMed]
48.Cui, X.; Breitenkamp, K.; Finn, M.; Lotz, M.; D’Lima, D.D. Direct Human Cartilage Repair Using Three-Dimensional Bioprinting Technology. Tissue Eng. Part A 2012, 18, 1304–1312. [CrossRef] [PubMed]
49.Marga, F.; Jakab, K.; Khatiwala, C.; Shephard, B.; Dorfman, S.; Forgacs, G. Organ printing: A novel tissue engineering paradigm. In Proceedings of the 5th European Conference of the International Federation for Medical and Biological Engineering, Budapest, Hungary, 14–18 September 2011; pp. 27–30.
50.Marga, F.; Jakab, K.; Khatiwala, C.; Shepherd, B.; Dorfman, S.; Hubbard, B.; Colbert, S.; Gabor, F. Toward engineering functional organ modules by additive manufacturing. Biofabrication 2012, 4, 022001. [CrossRef] [PubMed]
51.Mironov, V.; Kasyanov, V.; Markwald, R.R. Organ printing: From bioprinter to organ biofabrication line. Curr. Opin. Biotechnol. 2011, 22, 667–673. [CrossRef] [PubMed]
52.Kolesky, D.B.; Homan, K.A.; Skylar-Scott, M.A.; Lewis, J.A. Three-dimensional bioprinting of thick vascularized tissues. Proc. Natl. Acad. Sci. USA 2016, 113, 3179–3184. [CrossRef] [PubMed]
53.Chang, R.; Nam, J.; Sun, W. E ects of dispensing pressure and nozzle diameter on cell survival from solid freeform fabrication-based direct cell writing. Tissue Eng. Part A 2008, 14, 41–48. [CrossRef] [PubMed]
54.Duan, B.; Hockaday, L.A.; Kang, K.H.; Butcher, J.T. 3D bioprinting of heterogeneous aortic valve conduits with alginate/gelatin hydrogels. J. Biomed. Mater. Res. A 2013, 101, 1255–1264. [CrossRef]
55.Chang, R.; Nam, J.; Sun, W. Direct Cell Writing of 3D Microorgan for In Vitro Pharmacokinetic Model. Tissue Eng. Part C Methods 2008, 14, 157–166. [CrossRef] [PubMed]
56.Widmaier, E.P.; Ra , H.; Strang, K.T. Vander’s Human Physiology; McGraw-Hill Education: Boston, NY, USA, 2010.
57.Betts, J.G.; Desaix, P.; Johnson, J.E.; Korol, O.; Kruse, D.; Poe, B.; Womble, M.D. Anatomy & Physiology; Rice University: Houston, TX, USA, 2013.
58.Eguchi, S.; Chen, S.C.; Rozga, J.; Demetriou, A.A. Tissue Engineering: Liver. In Yearbook of Cell and Tissue Transplantation 1996–1997; Kluwer Academic Publishers: Dordrecht, The Netherlands, 1996; pp. 247–252. [CrossRef]
59.Tayyeb, A.; Azam, F.; Nisar, R.; Nawaz, R.; Qaisar, U.; Ali, G. Regenerative Medicine in Liver Cirrhosis: Promises and Pitfalls. In Liver Cirrhosis—Update and Current Challenges; BoD Publisher: Norderstedt, Germany, 2017. [CrossRef]
60.Khetani, S.R.; Bhatia, S.N. Microscale culture of human liver cells for drug development. Nat. Biotechnol. 2008, 26, 120–126. [CrossRef] [PubMed]
101
Bioengineering 2019, 6, 95
61.Hewitt, N.J.; Gómez Lechón, M.J.; Houston, J.B.; Hallifax, D.; Brown, H.S.; Maurel, P.; Kenna, J.G.; Gustavsson, L.; Lohmann, C.; Skonberg, C.; et al. Primary hepatocytes: Current understanding of the regulation of metabolic enzymes and transporter proteins, and pharmaceutical practice for the use of hepatocytes in metabolism, enzyme induction, transporter, clearance, and hepatotoxicity studies. Drug Metab. Rev. 2007, 39, 159–234. [CrossRef] [PubMed]
62.Gri th, L.G.; Wu, B.; Cima, M.J.; Powers, M.J.; Chaignaud, B.; Vacanti, J.P. In vitro organogenesis of liver tissue. Ann. N. Y. Acad. Sci. 1997, 831, 382–397. [CrossRef] [PubMed]
63.Takayama, K.; Kawabata, K.; Nagamoto, Y.; Kishimoto, K.; Tashiro, K.; Sakurai, F.; Tachibana, M.; Kanda, K.; Hayakawa, T.; Furue, M.K.; et al. 3D spheroid culture of hESC/hiPSC-derived hepatocyte-like cells for drug toxicity testing. Biomaterials 2013, 34, 1781–1789. [CrossRef] [PubMed]
64.Gaskell, H.; Sharma, P.; Colley, H.E.; Murdoch, C.; Williams, D.P.; Webb, S.D. Characterization of a functional C3A liver spheroid model. Toxicol. Res. (Camb.) 2016, 5, 1053–1065. [CrossRef]
65.Ho, C.T.; Lin, R.Z.; Chen, R.J.; Chin, C.K.; Gong, S.E.; Chang, H.Y.; Peng, H.L.; Hsu, L.; Yew, T.R.; Chang, S.F.; et al. Liver-cell patterning Lab Chip: Mimicking the morphology of liver lobule tissue. Lab Chip 2013, 13, 3578–3587. [CrossRef]
66.Berger, D.R.; Ware, B.R.; Davidson, M.D.; Allsup, S.R.; Khetani, S.R. Enhancing the functional maturity of iPSC-derived human hepatocytes via controlled presentation of cell-cell interactions in vitro. Hepatology 2014, 1, 1370–1381. [CrossRef]
67.Taub, R. Liver regeneration: From myth to mechanism. Nat. Rev. Mol. Cell Biol. 2004, 5, 836–847. [CrossRef]
68.Wang, X.; Yan, Y.; Pan, Y.; Xiong, Z.; Liu, H.; Cheng, J.; Liu, F.; Lin, F.; Wu, R.; Zhang, R.; et al. Generation of three-dimensional hepatocyte/gelatin structures with rapid prototyping system. Tissue Eng. 2006, 12, 83–90. [CrossRef]
69.Robbins, J.B.; Gorgen, V.; Min, P.; Shepherd, B.R.; Presnell, S.C. A novel in vitro three dimensional bioprinted liver tissue system for drug development. FASEB J. 2013, 27, 812–872.
70.Zein, N.N.; Hanouneh, I.A.; Bishop, P.D.; Samaan, M.; Eghtesad, B.; Quintini, C.; Miller, C.; Yerian, L.; Klatte, R. Three-dimensional print of a liver for preoperative planning in living donor liver transplantation. Liver Transpl. 2013, 19, 1304–1310. [CrossRef] [PubMed]
71.Nguyen, D.G.; Funk, J.; Robbins, J.B.; Crogan-Grundy, C.; Presnell, S.C.; Singer, T.; Roth, A.B. Bioprinted 3D primary liver tissues allow assessment of organ-level response to clinical drug induced toxicity in vitro. PLoS ONE 2016, 11, 0158674. [CrossRef] [PubMed]
72.Leva, V.; Chatzipetrou, M.; Alexopoulos, L.; Tzeranis, D.S.; Zergioti, I. Direct Laser Printing of Liver Cells on Porous Collagen Sca olds. JLMN J. Laser Micro Nanoeng. 2018, 13, 234–237. [CrossRef]
73.Arai, K.; Yoshida, T.; Okabe, M.; Goto, M.; Mir, T.A.; Soko, C.; Tsukamoto, Y.; Akaike, T.; Nikaido, T.; Zhou, K.; et al. Fabrication of 3D-culture platform with sandwich architecture for preserving liver-specific functions of hepatocytes using 3D bioprinter. J. Biomed. Mater. Res. Part A 2017, 105, 1583–1592. [CrossRef] [PubMed]
74.Matsusaki, M.; Sakaue, K.; Kadowaki, K.; Akashi, M. Three dimensional human tissue chips fabricated by rapid and automatic inkjet cell printing. Adv. Healthc. Mater. 2013, 2, 534–539. [CrossRef] [PubMed]
75.Kim, Y.; Kang, K.; Jeong, J.; Paik, S.S.; Kim, J.S.; Park, S.A.; Kim, W.D.; Park, J.; Choi, D. Three-dimensional (3D) printing of mouse primary hepatocytes to generate 3D hepatic structure. Ann. Surg. Treat. Res. 2017, 92, 67–72. [CrossRef] [PubMed]
76.Lee, J.W.; Choi, Y.J.; Yong, W.J.; Pati, F.; Shim, J.H.; Kang, K.S.; Kang, I.H.; Park, J.; Cho, D.W. Development of a 3D cell printed construct considering angiogenesis for liver tissue engineering. Biofabrication 2016, 8, 015007. [CrossRef]
77.Skardal, A.; Devarasetty, M.; Kang, H.W.; Mead, I.; Bishop, C.; Shupe, T.; Lee, S.J.; Jackson, J.; Yoo, J.; Soker, S. A hydrogel bioink toolkit for mimicking native tissue biochemical and mechanical properties in bioprinted tissue constructs. Acta Biomater. 2015, 25, 24–34. [CrossRef]
78.Mazzocchi, A.; Devarasetty, M.; Huntwork, R.C.; Soker, S.; Skardal, A. Optimization of collagen type I-hyaluronan hybrid bioink for 3D bioprinted liver microenvironments. Biofabrication 2018, 11, 015003. [CrossRef]
79.Lee, H.; Han, W.; Kim, H.; Ha, D.H.; Jang, J.; Kim, B.S.; Cho, D.W. Development of Liver Decellularized Extracellular Matrix Bioink for Three-Dimensional Cell Printing-Based Liver Tissue Engineering. Biomacromolecules 2017, 18, 1229–1237. [CrossRef] [PubMed]
102
Bioengineering 2019, 6, 95
80.Hiller, T.; Berg, J.; Elomaa, L.; Röhrs, V.; Ullah, I.; Schaar, K.; Dietrich, A.C.; Al-Zeer, M.A.; Kurtz, A.; Hocke, A.C.; et al. Generation of a 3D Liver Model Comprising Human Extracellular Matrix in an Alginate/Gelatin-Based Bioink by Extrusion Bioprinting for Infection and Transduction Studies. Int. J. Mol. Sci. 2018, 19, 3129. [CrossRef] [PubMed]
81.Faulkner-Jones, A.; Greenhough, S.; King, J.A.; Gardner, J.; Courtney, A.; Shu, W. Development of a valve-based cell printer for the formation of human embryonic stem cell spheroid aggregates. Biofabrication 2013, 5, 015013. [CrossRef] [PubMed]
82.Faulkner-Jones, A.; Fyfe, C.; Cornelissen, D.J.; Gardner, J.; King, J.; Courtney, A.; Shu, W. Bioprinting of human pluripotent stem cells and their directed di erentiation into hepatocyte-like cells for the generation of mini-livers in 3D. Biofabrication 2015, 7, 044102. [CrossRef] [PubMed]
83.Lei, M.; Wang, X. Biodegradable Polymers and Stem Cells for Bioprinting. Molecules 2016, 21, 539. [CrossRef] [PubMed]
84.Kim, Y.; Kang, K.; Yoon, S.; Kim, J.S.; Park, S.A.; Kim, W.D.; Lee, S.B.; Ryu, K.Y.; Jeong, J.; Choi, D. Prolongation of liver-specific function for primary hepatocytes maintenance in 3D printed architectures. Organogenesis 2018, 14, 1–12. [CrossRef] [PubMed]
85.Orlando, G.; Baptista, P.; Birchall, M.; Coppi, P.D.; Farney, A.; Guimaraes-Souza, N.K.; Opara, E.; Rogers, J.; Seliktar, D.; Shapira-Schweitzer, K.; et al. Regenerative medicine as applied to solid organ transplantation: Current status and future challenges. Transpl. Int. 2011, 24, 223–232. [CrossRef] [PubMed]
86.Chaudhari, P.; Tian, L.; Deshmukh, A.; Jang, Y.-Y. Expression kinetics of hepatic progenitor markers in cellular models of human liver development recapitulating hepatocyte and biliary cell fate commitment. Exp. Biol. Med. 2016, 241, 1653–1662. [CrossRef]
87.Maza, G.; Rombouts, K.; Hall, A.R.; Urbani, L.; Luong, T.V.; Al-Akkad, W.; Longato, L.; Brown, D.; Maghsoudlou, P.; Dhillon, A.P.; et al. Decellularized human liver as a natural 3D-sca old for liver bioengineering and transplantation. Sci. Rep. 2015, 5, 13079. [CrossRef]
88.Hussein, K.H.; Park, K.M.; Ghim, J.H.; Yang, S.R.; Woo, H.M. Three dimensional culture of HepG2 liver cells on a rat decellularized liver matrix for pharmacological studies. J. Biomed. Mater. Res. Part B Appl. Biomater. 2016, 104, 263–273. [CrossRef]
89.Kizawa, H.; Nagao, E.; Shimamura, M.; Zhang, G.; Torii, H. Sca old-free 3D bio-printed human liver tissue stably maintains metabolic functions useful for drug discovery. Biochem. Biophys. Rep. 2017, 10, 186–191. [CrossRef] [PubMed]
90.Schepers, A.; Li, C.; Chhabra, A.; Seney, B.T.; Bhatia, S. Engineering a perfusable 3D human liver platform from iPS cells. Lab Chip 2016, 16, 2644–2653. [CrossRef] [PubMed]
91.Shamir, E.R.; Ewald, A.J. Three-dimensional organotypic culture: Experimental models of mammalian biology and disease. Nat. Rev. Mol. Cell Biol. 2014, 15, 647. [CrossRef] [PubMed]
92.Qian, X.; Nguyen, H.N.; Song, M.M.; Hadiono, C.; Ogden, S.C.; Hammack, C.; Yao, B.; Hamersky, G.R.; Jacob, F.; Zhong, C.; et al. Brain-Region-Specific Organoids Using Mini-bioreactors for Modeling ZIKV Exposure. Cell 2016, 165, 1238–1254. [CrossRef] [PubMed]
93.Frey, O.; Misun, P.M.; Fluri, D.A.; Hengstler, J.G.; Hierlemann, A. Reconfigurable microfluidic hanging drop network for multi-tissue interaction and analysis. Nat. Commun. 2014, 5, 4250. [CrossRef] [PubMed]
94.Tung, Y.C.; Hsiao, A.Y.; Allen, S.; Torisawa, Y.S.; Ho, M.; Takayama, S. High-throughput 3D spheroid culture and drug testing using a 384 hanging drop array. Analyst 2011, 136, 473–478. [CrossRef] [PubMed]
95.Norona, L.M.; Nguyen, D.G.; Gerber, D.A.; Presnell, S.C.; Lecluyse, E.L. Editor’s Highlight: Modeling Compound-Induced Fibrogenesis In Vitro Using Three-Dimensional Bioprinted Human Liver Tissues. Toxicol. Sci. 2016, 154, 354–367. [CrossRef] [PubMed]
96.Kwon, R.Y.; Meays, D.R.; Tang, W.J.; Frangos, J.A. Microfluidic Enhancement of Intramedullary Pressure Increases Interstitial Fluid Flow and Inhibits Bone Loss in Hindlimb Suspended Mice. J. Bone Miner. Res. 2010, 25, 1798–1807. [CrossRef] [PubMed]
97.Chung, B.G.; Flanagan, L.A.; Rhee, S.W.; Schwartz, P.H.; Lee, A.P.; Monuki, E.S.; Jeon, N.L. Human neural stem cell growth and di erentiation in a gradient-generating microfluidic device. Lab Chip 2005, 5, 401–406. [CrossRef]
98.Andersson, H.; Van Den Berg, A. Microfabrication and microfluidics for tissue engineering: State of the art and future opportunities. Lab Chip 2004, 4, 98–103. [CrossRef]
103
Bioengineering 2019, 6, 95
99.Takayama, S.; Ostuni, E.; LeDuc, P.; Naruse, K.; Ingber, D.E.; Whitesides, G.M. Subcellular positioning of small molecules. Nature 2001, 411, 1016. [CrossRef] [PubMed]
100.Saxena, V.; Nyberg, L.; Pauly, M.; Dasgupta, A.; Nyberg, A.; Piasecki, B.; Winston, B.; Redd, J.; Ready, J.; Terrault, N.A. Safety and E cacy of Simeprevir/Sofosbuvir in Hepatitis C-Infected Patients with Compensated and Decompensated Cirrhosis. Hepatology 2015, 62, 715–725. [CrossRef] [PubMed]
101.Geerts, A. History, heterogeneity, developmental biology, and functions of quiescent hepatic stellate cells. Semin. Liver Dis. 2001, 21, 311–336. [CrossRef] [PubMed]
102.Prot, J.M.; Aninat, C.; Griscom, L.; Razan, F.; Guillouzo, C.G.; Corlu, A.; Brochot, C.; Legallais, C.; Leclerc, E. Improvement of HepG2/C3a cell functions in a microfluidic biochip. Biotechnol. Bioeng. 2011, 108, 1704–1715. [CrossRef] [PubMed]
103.Kim, C.; Kasuya, J.; Jeon, J.; Chung, S.; Kamm, R.D. A quantitative microfluidic angiogenesis screen for studying anti-angiogenic therapeutic drugs. Lab Chip 2015, 15, 301–310. [CrossRef] [PubMed]
104.Korin, N.; Kanapathipillai, M.; Matthews, B.D.; Crescente, M.; Brill, A.; Mammoto, T.; Ghosh, K.; Jurek, S.; Bencherif, S.A.; Bhatta, D.; et al. Shear-activated nanotherapeutics for drug targeting to obstructed blood vessels. Science 2012, 337, 738–742. [CrossRef] [PubMed]
105.Kang, Y.B.A.; Sodunke, T.R.; Lamontagne, J.; Cirillo, J.; Rajiv, C.; Bouchard, M.J.; Noh, M. Liver sinusoid on a chip: Long-term layered co-culture of primary rat hepatocytes and endothelial cells in microfluidic platforms. Biotechnol. Bioeng. 2015, 112, 2571–2582. [CrossRef] [PubMed]
106.Zhou, Q.; Patel, D.; Kwa, T.; Haque, A.; Matharu, Z.; Stybayeva, G.; Gao, Y.; Diehl, A.M.; Revzin, A. Liver injury-on-a-chip: Microfluidic co-cultures with integrated biosensors for monitoring liver cell signaling during injury. Lab Chip 2015, 15, 4467–4478. [CrossRef]
107.Kietzmann, T. Metabolic zonation of the liver: The oxygen gradient revisited. Redox Biol. 2017, 11, 622–630. [CrossRef] [PubMed]
108.Allen, J.W.; Bhatia, S.N. Formation of steady-state oxygen gradients in vitro:Application to liver zonation.
Biotechnol. Bioeng. 2003, 82, 253–262. [CrossRef]
109.McCarty, W.J.; Usta, O.B.; Yarmush, M.L. A microfabricated platform for generating physiologically-relevant hepatocyte zonation. Sci. Rep. 2016, 6, 26868. [CrossRef] [PubMed]
110.Bhise, N.S.; Manoharan, V.; Massa, S.; Tamayol, A.; Ghaderi, M.; Miscuglio, M.; Lang, Q.; Zhang, Y.S.; Shin, S.R.; Calzone, G.; et al. A liver-on-a-chip platform with bioprinted hepatic spheroids. Biofabrication 2016, 8, 014101. [CrossRef] [PubMed]
111.Chang, R.; Emami, K.; Wu, H.; Sun, W. Biofabrication of a three-dimensional liver micro-organ as anin vitrodrug metabolism model. Biofabrication 2010, 2, 045004. [CrossRef] [PubMed]
112.Novik, E.; Maguire, T.J.; Chao, P.; Cheng, K.C.; Yarmush, M.L. A microfluidic hepatic coculture platform for cell-based drug metabolism studies. Biochem. Pharmacol. 2010, 79, 1036–1044. [CrossRef] [PubMed]
113.Cho, C.H.; Park, J.; Tilles, A.W.; Berthiaume, F.; Toner, M.; Yarmush, M.L. Layered patterning of hepatocytes in co-culture systems using microfabricated stencils. Biotechniques 2010, 48, 47–52. [CrossRef] [PubMed]
114.Vernetti, L.A.; Senutovitch, N.; Boltz, R.; DeBiasio, R.; Shun, T.Y.; Gough, A.; Taylor, D.L. A human liver microphysiology platform for investigating physiology, drug safety, and disease models. Exp. Biol. Med. (Maywood) 2016, 241, 101–114. [CrossRef] [PubMed]
115.Wong, S.F.; No, D.Y.; Choi, Y.Y.; Kim, D.S.; Chung, B.G.; Lee, S.H. Concave microwell based size-controllable hepatosphere as a three-dimensional liver tissue model. Biomaterials 2011, 32, 8087–8096. [CrossRef] [PubMed]
116.Holt, E.; Lunde, P.K.; Sejersted, O.M.; Christensen, G. Electrical stimulation of adult rat cardiomyocytes in culture improves contractile properties and is associated with altered calcium handling. Basic Res. Cardiol. 1997, 92, 289–298. [CrossRef] [PubMed]
117.Karageorgiou, V.; Kaplan, D. Porosity of 3D biomaterial sca olds and osteogenesis. Biomaterials 2005, 26, 5474–5491. [CrossRef] [PubMed]
118.Lee, K.W.; Wang, S.; Dadsetan, M.; Yaszemski, M.J.; Lu, L. Enhanced cell ingrowth and proliferation through three-dimensional nanocomposite sca olds with controlled pore structures. Biomacromolecules 2010, 11, 682–689. [CrossRef]
119.Nguyen, A.K.; Narayan, R.J. Two-photon polymerization for biological applications. Mater. Today 2017, 20, 314–322. [CrossRef]
104
Bioengineering 2019, 6, 95
120.Ovsianikov, A.; Gruene, M.; Pflaum, M.; Koch, L.; Maiorana, F.; Wilhelmi, M.; Haverich, A.; Chichkov, B. Laser printing of cells into 3D sca olds. Biofabrication 2010, 2, 014104. [CrossRef] [PubMed]
121.Rekštyte, S.; Kaziulionyte, E.; Balciunas, E.; Kaškelyte, D.; Malinauskas, M. Direct laser fabrication of composite material 3D microstructured sca olds. JLMN J. Laser Micro Nanoeng. 2014, 9. [CrossRef]
122.Ovsianikov, A.; Deiwick, A.; Van Vlierberghe, S.; Pflaum, M.; Wilhelmi, M.; Dubruel, P.; Chichkov, B. Laser Fabrication of 3D Gelatin Sca olds for the Generation of Bioartificial Tissues. Materials 2011, 4, 288–299. [CrossRef] [PubMed]
123.Zheng, Y.C.; Zhao, Y.Y.; Zheng, M.L.; Chen, S.L.; Liu, J.; Jin, F.; Dong, X.Z.; Zhao, Z.S.; Duan, X.M. Cucurbit uril-Carbazole Two-Photon Photoinitiators for the Fabrication of Biocompatible Three-Dimensional Hydrogel Sca olds by Laser Direct Writing in Aqueous Solutions. ACS Appl. Mater. Interfaces 2019, 11, 1782–1789. [CrossRef] [PubMed]
124.Koroleva, A.; Gittard, S.; Schlie, S.; Deiwick, A.; Jockenhoevel, S.; Chichkov, B. Fabrication of fibrin sca olds with controlled microscale architecture by a two-photon polymerization–micromolding technique. Biofabrication 2012, 4, 015001. [CrossRef] [PubMed]
125.Du, Y.; Liu, H.; Yang, Q.; Wang, S.; Wang, J.; Ma, J.; Noh, I.; Mikos, A.G.; Zhang, S. Selective laser sintering sca old with hierarchical architecture and gradient composition for osteochondral repair in rabbits. Biomaterials 2017, 137, 37–48. [CrossRef] [PubMed]
126.Lohfeld, S.; Tyndyk, M.A.; Cahill, S.; Flaherty, N.; Barron, V.; McHugh, P.E. A method to fabricate small features on sca olds for tissue engineering via selective laser sintering. J. Biomed. Sci. Eng. 2010, 3, 138–147. [CrossRef]
127.Negro, A.; Cherbuin, T.; Lutolf, M.P. 3D Inkjet Printing of Complex, Cell-Laden Hydrogel Structures. Sci. Rep. 2018, 8, 17099. [CrossRef] [PubMed]
128.Mi, S.; Yang, S.; Liu, T.; Du, Z.; Xu, Y.; Li, B.; Sun, W. A Novel Controllable Cell Array Printing Technique on Microfluidic Chips. IEEE Trans. Biomed. Eng. 2019, 66, 2512–2520. [CrossRef] [PubMed]
129.Derby, B. Bioprinting: Inkjet printing proteins and hybrid cell-containing materials and structures. J. Mater. Chem. 2008, 18, 5717–5721. [CrossRef]
130.Saunders, R.E.; Derby, B. Inkjet printing biomaterials for tissue engineering: Bioprinting. Int. Mater. Rev. 2014, 59, 430–448. [CrossRef]
131.Roth, E.A.; Xu, T.; Das, M.; Gregory, C.; Hickman, J.J.; Boland, T. Inkjet printing for high-throughput cell patterning. Biomaterials 2004, 25, 3707–3715. [CrossRef] [PubMed]
132.Xu, T.; Baicu, C.; Aho, M.; Zile, M.; Boland, T. Fabrication and characterization of bio-engineered cardiac pseudo tissues. Biofabricatioin 2009, 1, 035001. [CrossRef] [PubMed]
133.Cui, X.; Boland, T. Human microvasculature fabrication using thermal inkjet printing technology. Biomaterials 2009, 30, 6221–6227. [CrossRef] [PubMed]
134.Zhang, C.; Wen, X.; Vyavahare, N.R.; Boland, T. Synthesis and characterization of biodegradable elastomeric polyurethane sca olds fabricated by the inkjet tech-nique. Biomaterials 2008, 29, 3781–3791. [CrossRef] [PubMed]
135.Delaney, J.T.; Liberski, A.R.; Perelaer, J.; Schubert, U.S. Reactive inkjet printing of calcium alginate hydrogel porogens—A new strategy to open-pore structured matrices with controlled geometry. Soft Matter 2010, 6, 866–869. [CrossRef]
136.Di Biase, M.; Saunders, R.E.; Tirelli, N.; Derby, B. Inkjet printing and cell seeding thermoreversible photocurable gel structures. Soft Matter 2011, 7, 2639–2646. [CrossRef]
137.Xu, T.; Gregory, C.A.; Molnar, P.; Cui, X.; Jalota, S.; Bhaduri, S.B.; Boland, T. Viability and electrophysiology of neural cell structures generated by the inkjet printing method. Biomaterials 2006, 27, 3580–3588. [CrossRef] [PubMed]
138.Lewis, P.L.; Green, R.M.; Shah, R.N. 3D-printed gelatin sca olds of di ering pore geometry modulate hepatocyte function and gene expression. Acta Biomater. 2018, 69, 63–70. [CrossRef] [PubMed]
139.Kang, K.; Kim, Y.; Jeon, H.; Lee, S.B.; Kim, J.S.; Park, S.A.; Kim, W.D.; Yang, H.M.; Kim, S.J.; Jeong, J.; et al. Three-Dimensional Bioprinting of Hepatic Structures with Directly Converted Hepatocyte-Like Cells. Tissue Eng. Part A 2018, 24, 576–583. [CrossRef] [PubMed]
105

Bioengineering 2019, 6, 95
140.Jeon, H.; Kang, K.; Park, S.A.; Kim, W.D.; Paik, S.S.; Lee, S.H.; Jeong, J.; Choi, D. Generation of Multilayered 3D Structures of HepG2 Cells Using a Bio-printing Technique. Gut Liver 2017, 11, 121–128. [CrossRef] [PubMed]
141.Gong, H.; Agustin, J.; Wootton, D.; Zhou, J.G. Biomimetic design and fabrication of porous chitosan–gelatin liver sca olds with hierarchical channel network. J. Mater. Sci. Mater. Med. 2014, 25, 113–120. [CrossRef] [PubMed]
©2019 by the authors. Licensee MDPI, Basel, Switzerland. This article is an open access article distributed under the terms and conditions of the Creative Commons Attribution (CC BY) license (http://creativecommons.org/licenses/by/4.0/).
106
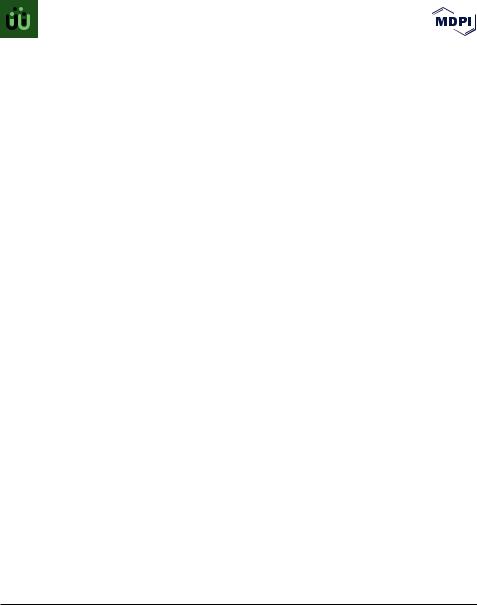
bioengineering
Article
3D Printing for Bio-Synthetic Biliary Stents
Christen J. Boyer 1,2, Moheb Boktor 3, Hrishikesh Samant 3, Luke A. White 1, Yuping Wang 4, David H. Ballard 5, Robert C. Huebert 6, Jennifer E. Woerner 2, Ghali E. Ghali 2 and
Jonathan S. Alexander 1,*
1Molecular and Cellular Physiology, Health Sciences Center, Louisiana State University, Shreveport, LA 71103, USA; cboye2@lsuhsc.edu (C.J.B.); lwhit9@lsuhsc.edu (L.A.W.)
2Oral and Maxillofacial Surgery, Health Sciences Center, Louisiana State University, Shreveport, LA 71103,
USA; jwoern@lsuhsc.edu (J.E.W.); GGhali@lsuhsc.edu (G.E.G.)
3Gastroenterology and Hepatology, Health Sciences Center, Louisiana State University, Shreveport, LA 71103,
USA; mbokto@lsuhsc.edu (M.B.); hsaman@lsuhsc.edu (H.S.)
4Obstetrics and Gynecology, Health Sciences Center, Louisiana State University, Shreveport, LA 71103, USA; YWang1@lsuhsc.edu
5Mallinckrodt Institute of Radiology, School of Medicine, Washington University, St. Louis, MO 63110, USA;
davidballard@wustl.edu
6Gastroenterology and Hepatology, Mayo Clinic, Rochester, MN 55905, USA; Huebert.Robert@mayo.edu * Correspondence: jalexa@lsuhsc.edu; Tel.: +1-318-675-4151; Fax: +1-318-675-6005
Received: 8 January 2019; Accepted: 6 February 2019; Published: 9 February 2019
Abstract: Three-dimensional (3D) printing is an additive manufacturing method that holds great potential in a variety of future patient-specific medical technologies. This project validated a novel crosslinked polyvinyl alcohol (XL-PVA) 3D printed stent infused with collagen, human placental mesenchymal stem cells (PMSCs), and cholangiocytes. The biofabrication method in the present study examined 3D printing and collagen injection molding for rapid prototyping of customized living biliary stents with clinical applications in the setting of malignant and benign bile duct obstructions. XL-PVA stents showed hydrophilic swelling and addition of radiocontrast to the stent matrix improved radiographic opacity. Collagen loaded with PMSCs contracted tightly around hydrophilic stents and dense choloangiocyte coatings were verified through histology and fluorescence microscopy. It is anticipated that design elements used in these stents may enable appropriate stent placement, provide protection of the stent-stem cell matrix against bile constituents, and potentially limit biofilm development. Overall, this approach may allow physicians to create personalized bio-integrating stents for use in biliary procedures and lays a foundation for new patient-specific stent fabrication techniques.
Keywords: 3D printing; hepatobiliary stent; tissue engineering; medical device; stem cells; personalized medicine
1. Introduction
Biliary obstruction can be caused by both benign and malignant conditions including iatrogenic bile duct injury and chronic pancreatitis as well as cholangiocarcinoma and pancreatic cancer [1,2]. Relief from such obstructions can help minimize obstructive jaundice and reduce the risk of cholangitis [3,4]. The endoscopic placement of hepatobiliary stents was first performed in 1980 to restore biliary flow [5,6]. Since then, such endoscopic techniques have become favored alternatives to surgery due to their less invasive nature and decreased morbidity associated with these procedures [7].
Several types of stents have been developed over the years, each with their own advantages and disadvantages. Plastic stents were used first and today are still the most commonly used device [5,6]. In the late 1980s, self-expanding metal stents were first used in the biliary tract [8–10]. Compared
Bioengineering 2019, 6, 16; doi:10.3390/bioengineering6010016107 |
www.mdpi.com/journal/bioengineering |
Bioengineering 2019, 6, 16
to plastic stents, self-expanding metal stents have decreased mortality as well as the number of re-interventions, however, self-expanding metal stents are more expensive [4].
More recently, drug-eluting stents have been developed as an approach to maintain stent patency [11]. Both plastic and metal stents with anti-reflux valves have been explored as a means to decrease cholangitis and increase patency [12]. The use of biodegradable biliary stents has also been explored. In many clinical scenarios, biliary stents are only needed temporarily [13,14]. A biodegradable stent that dissolves allowing its remnants to be expelled into the duodenum would eliminate the need for a secondary device removal procedure, which would increase the risk for recurrent biliary occlusion or stenosis.
Despite many advances in stent development, one major problem that remains is the progressive loss of stent patency over time. Several factors contribute to this loss of patency. It is widely accepted that biofilm formation on the stent is a common cause of stent failure. Bacteria gain access to biliary stents by migrating from either the portal venous system or through the sphincter of Oddi [15]. Microorganisms may then adhere to the stent and begin to proliferate and secrete exopolysaccharides to form biofilms that resist antibiotic treatments. Biofilm creation, in turn, is thought to contribute to biliary “sludge” formation that ultimately leads to the loss of stent patency [16].
One area that remains unexplored is the development and use of 3D printed hepatobiliary stents. 3D printing is an emerging technology in medicine, and it would allow for the rapid production of custom medical products that are relatively inexpensive [17]. The application of 3D printed stents has been explored for use in many diseases. Several examples include the use of 3D printed vascular stents for percutaneous coronary intervention, airway stents for the treatment of central airway obstruction, esophageal stents for palliative care in inoperable esophageal malignancies, and ureteral stents which facilitate urine drainage from the kidney [18–21].
Cytocompatible, hydrophilic, and drug-deliverable 3D printed materials and techniques have been recently explored and hold great promise in cell culture models, medical devices, and tissue-engineered substrates [22–25]. Such materials include 3D printed polyvinyl alcohol (PVA), a water soluble polymer. PVA filaments demonstrate favorable 3D printability using fused deposition modeling (FDM) techniques which can be post-print crosslinked (XL) to form stable 3D hydrogels [26]. The 3D printed crosslinked polyvinyl alcohol (XL-PVA) method is capable of binding biomolecules, drugs, and cells of interest due to the molecular complexes formed during crosslinking. Such materials have applications in a variety of medical devices and wound care substrates. In this study, the XL-PVA 3D printing method was coupled with collagen injection molding to create hepatobiliary stents which have been engineered to support engraftment with human placental stem cells and cholangiocytes.
2. Materials and Methods
2.1. PVA Stent Fabrication and Crosslinking
Porous tubular stents (25 mm length, 5 mm external diameter, and 3 mm internal diameter) were created with free computer aided design (CAD) software (Tinker-CAD, AutoDesk, San Francisco, CA, USA). 96 square pores (1 mm × 1 mm) were created in the stent design with 1 mm spacing between each pore. PVA filaments (AquaSolve™, Formfutura, Nijmegen, Netherlands, 1.75 mm diameter) were 3D printed into the designed stent pattern at 201 ◦C using a consumer grade 3D printed (MakerBot Replicator desktop 3D printer, MakerBot Industries LLC, Brooklyn, NY, USA) with supports turned off and raft turned on. PVA 3D printed stents were immersed in distilled water briefly to fuse layers, and cross-linked (XL) by placing the stents in a gas vapor desiccator with two separate containers containing 20 mL of 6.25% glutaraldehyde (GA) (EMD Millipore Corporation, Darmstadt, Germany) and 10 mL of concentrated hydrochloric acid (HCl) (Fisher Scientific, Hampton, NH, USA) at 42 ◦C for 24 h. XL-PVA stents were next rinsed extensively in distilled water and soaked in 70% ethanol for 24 h. XL-PVA stents were rinsed again and placed in 1X phosphate buffer solution (PBS) for storage.
108
Bioengineering 2019, 6, 16
2.2. Collagen Injection Mold Chamber Fabrication
Cylindrical injection mold chambers and stent lumen maturation plugs were designed with free CAD software (Tinker-CAD) and 3D printed on a consumer grade stereolithography 3D printer (Formlabs, Form2, Somerville, MA, USA) using flexible resin (Formlabs) with supports turned on. 3D printed parts were rinsed in 70% isopropyl alcohol (Form Wash, Formlabs) and then cured at 60 ◦C with shortwave ultraviolet light (UV) for 1 h (Form Cure, Formlabs). Injection mold chambers and maturation plugs were next rinsed in 70% ethanol followed by a 1× PBS wash in a sterile tissue culture hood and stored there until used in experiments.
2.3. Barium Sulfate Coating and X-Ray Imaging
Polycaprolactone (PCL) (Sigma Aldrich, St. Louis, MO, USA) (molecular weight 80,000) and E-Z-HD™ barium sulfate (BS) (E-Z-EM Canada Inc., Quebec, Canada) were mixed in chloroform (CF) (Sigma Aldrich) to form 10% PCL:10% BS:80% CF mixture. Hydrated XL-PVA stents were dipped in the PCL-BS-CF mixtures on each tip’s end and the chloroform was allowed to evaporate before placing the stents back into 1× PBS. Final dry PCL-BS coatings on stent tips contained 50% PCL:50% BS. XL-PVA stents and versions with PCL-BS coatings were imaged with an OEC 9900 Elite C-Arm System X-ray (General Electric, Fairfield, CT, USA) in 10 mL of 1× PBS.
2.4. XL-PVA Swelling Analysis
For swelling studies, XL-PVA pieces (n = 5) were hydrated overnight in 1X PBS and dehydrated. Each hydrated and dehydrated form was weighed to obtain average weights. To calculate mass change percentage ( M%), the average hydrated weight (M1) was subtracted from the average dry weight (M2), then divided by the hydrated weight (M1) and multiplied by 100. The mass swelling ratio was calculated by taking the ratio between the average mass of the hydrated XL-PVA (M1) and the average mass of the dehydrated XL-PVA (M2), where M1 is divided by M2. The results were statistically analyzed by using Student’s t-test and the data were expressed as the mean ± standard deviation (SD). A p-value of <0.05 was considered statistically significant.
2.5. Collagen Preparation
Rat tail type 1 collagen matrices were prepared by a modification of the protocol previously published by Benoit et al. [27]. Briefly, rat tail tendons were manually excised, washed with 100% isopropanol (Thermo Fisher Scientific, Waltham, MA, USA) and dissolved in sterile 4 mM acetic acid (Sigma Aldrich) for 24 h at 4 ◦C under constant agitation. Collagen solution was filtered through a 250 μm nylon filter (Spectrum Labs, Rancho Dominguez, CA, USA), centrifuged at 19× g for 20 min at 4 ◦C and snap frozen. Using a bench-top manifold freeze-dryer (Millrock Technology, Kingston, NY, USA), frozen aliquots were dried and stored at −20 ◦C for future use. Twenty-four hours prior to experiments, freeze-dried collagen was resolubilized in cold 0.012 M hydrochloric acid (HCl) (Sigma Aldrich) at 2.5 mg/mL final collagen concentration and incubated overnight at 4 ◦C with gentle agitation. On the day of the experiment, 0.8 mL of cold 5X PBS was added to 3.2 mL of dissolved collagen gel and the pH was titrated with 0.5 M sodium hydroxide (NaOH) (Sigma Aldrich) to 7.4.
2.6. Isolation of Mesenchymal Stem Cells
Human placentas were collected from normal term pregnancies. Collection of human placenta for mesenchymal stem cell isolation was approved by the Institutional Review Board (IRB) (approval number CR00001345_STUDY00000614). Human placental mesenchymal stem cells (PMSCs) were obtained by cultivation of microvilli after elimination of villous trophoblasts. The villous tissue was dissected from different cotyledons, excluding chorionic and basal plates. After rinsing with ice-cold PBS, the villous tissue was next digested with trypsin (0.125% trypsin solution (Sigma Aldrich) containing 0.1 mg/mL DNase I and 5 (Sigma Aldrich) mM MgCl2 (Sigma Aldrich) in Dulbecco’s
109