
Mechanical Properties of Ceramics and Composites
.pdf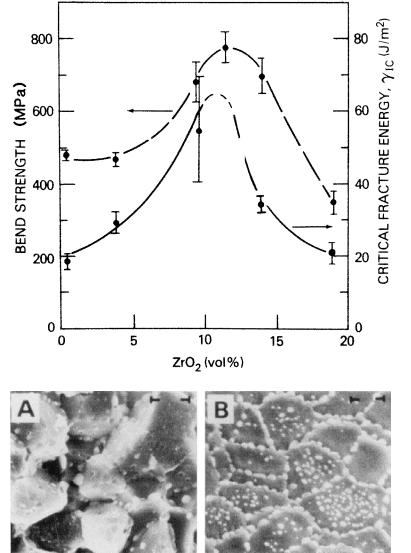
550 |
Chapter 9 |
FIGURE 9.6 Fracture toughness and strength data of Becher [25] for composites of an alumina matrix versus the volume percent of unstabilized ZrO2 particles. (A) and (B) are SEM photos of respectively a fracture and an as-fired surface showing the uniform microstructure, especially the particle size and distribution of the ZrO2 as a result of sol–gel composite processing (bars = 5 m). Note the similar trends of strength and toughness in contrast to Fig. 9.5. (From Ref. 24. Published with permission of Noyes Publications.)

Particle Dependence of Tensile Strength |
551 |
FIGURE 9.7 Schematic summary of the increases in strength of Al2O3-ZrO2 bodies as a function of ZrO2 and stabilizer content showing combined effects of reduced Al2O3 grain size and ZrO2 stabilized content.
Subsequent work, apparently first by Lange and Hirlinger [27] showed that dispersions of ZrO2 particles in Al2O3 powder compacts can significantly limit Al2O3 grain growth, resulting in a finer Al2O3 grain size. However, they reported that with uniform mixing at least 2.5 m/o ZrO2 was needed to control exaggerated grain growth (and more with less uniform mixing). Hori and colleagues [28,29] corroborated such grain growth benefits, even for lower levels of fine ZrO2 additions, and showed that this made useful increases in strengths of such bodies, despite some lowering of toughness. Thus they showed that the Al2O3 grain size dropped from 4 + to 2.5 m as the ZrO2 content went from 0 to 0.1 w/o (with a ZrO2 particle size of 0.2 + m) and decreased less rapidly as the ZrO2 content was further increased, reaching G 1.5 m at 5 w/o ZrO2 (with its particle size of 0.3 m). These composition and microstructure changes resulted in composite strengths increasing rapidly at lower additions, and then more slowly, i.e. starting from 310 MPa and reaching 570 MPa as the matrix
552 |
Chapter 9 |
grain size decreased, consistent with behavior of pure Al2O3 (Fig. 3.14), again showing the importance of matrix grain size on mechanical properties of ceramic composites. The strength increases occurred despite the (IF) toughness decreasing from 4.8 MPa·m1/2 with no ZrO2 to a minimum of 3.9 MPa·m1/2 at 1.1 w/o ZrO2, and then rising to 4.6 MPa·m1/2 at 5 w/o ZrO2, again showing basic differences in larger crack toughness behavior from that of strength. The collective mechanisms are schematically summarized in Figure 9.7.
The above effects of ZrO2 additions in limiting matrix grain growth are expected to impact most composites. Additional explicit evidence of this is provided by Nisida et al.’s [30] study of composites with up to 10 w/o of either fine tetragonal or cubic ZrO2 particles in MgO. While the ZrO2 particles coarsened (respectively from 0.3–0.4 to 0.4–1 + m) as ZrO2 content and densification temperatures increased, MgO G decreased up to threeand fivefold respectively for lower and higher temperature sintering. Resultant strengths were primarily determined by the resultant MgO G as shown by comparison to their own MgO without ZrO2, as well as general agreement with data of Figure 3.5, except for some tendency for deviation to less strength increases at the finer G with higher ZrO2 contents.
While the primary toughening mechanism in ZrO2 toughened bodies is transformation, microcracking can also occur and was proposed as a major source of toughening in ZTA composites [23,31–33]. Thus duplex designed microstructures using more stabilized, and higher volume fractions of, ZrO2 particles can result in substantial microcracking with propagation of large cracks and thus can give higher thermal shock resistance (Chap. 8, Sec. V.B, and Chap. 11, Sec. III.C). However, while this produces significant R-curve effects and increases fracture toughness and residual strengths after serious thermal shocks, initial strengths decreased substantially, e.g. from 1300–1700 MPa to 100–800 MPa [33].
Wang and Stevens [34] investigated Al2O3-ZrO2 composites of various microstructures using normal ZrO2 particle dispersions, dispersions of TZP particles, and combinations of these, i.e. duplex structures as above. Bodies sintered with dispersions of individual, fine ( 1 m) unstabilized ZrO2 particles showed modest strengths of 400 MPa decreasing slightly from 0 to 10 v/o ZrO2, dropping to 200 MPa at 12 v/o and then to 100 MPa at 20 v/o ZrO2, i.e. essentially a mirror image of their I toughness results (Chap. 8, Sec. V.B). Bodies with addition of both 2.5Y-TZP agglomerates (20–50 m) and the unstabilized ZrO2 particles, i.e. composites along the lines of Fig. 2.18 showed a modest linear increase in strength at 10 v/o, reaching 300 MPa at 40 v/o combined additions for sintered bodies and approximately double these values in hot pressed bodies. The increase from 97 to 100% of theoretical density for hot pressing versus sintering appears inadequate to explain the doubling of strength by hot pressing. Reductions of Al2O3 and ZrO2 grain and particle sizes to 1/2 the values in the
Particle Dependence of Tensile Strength |
553 |
sintered bodies (respectively 4 and 1 m) are probably also significant in increased strengths in hot pressed bodies, and some stabilization of the ZrO2 particles due to some probable reduction in hot pressing is also a possible factor, as is discussed below. While the trend of strengths to increase with v/o additions is consistent with the trends of their I toughnesses, the absolute values of their strengths and the extent of their change with v/o ZrO2 are clearly not consistent, since their toughness increased threefold. They attributed toughening of the composites with unstabilized particles alone to transformation and microcrack toughening, that with TZP agglomerated alone to transformation and crack deflection, and all of these with both types of addition. The indicated presence of microcracking with all additions is consistent with the limited strengths (especially those from < 100 to 300 MPa) and indicates that the combined composite is a probable manifestation of either, or probably both, of those sketched in Figures 8.1B and 8.2C.
The first of two additional points that should be noted is that while transformation of ZrO2 particles can be very effective for toughening and strengthening the bodies containing them, either cubic or monoclinic ZrO2 particles can contribute some toughening, strengthening, or both. Thus monoclinic particles can cause microcracking and resultant toughening or can toughen via crack deflections resulting from the substantial stress fields associated with their presence (without microcracking as in a ZnO matrix) as discussed in Chap. 8, Sec. V.B. The latter is a possibly more extreme example of a more general effect, namely the presence of ZrO2 particles in a matrix should provide toughening to the extent that they can impede crack propogation due to crack deflection, bowing, or other mechanisms with other nontransforming particles. This is consistent with the recognition that metastable or stable tetragonal ZrO2 precipitates (i.e. above the tetragonal–monoclinic transformation temperature) in cubic ZrO2 provide substantial toughening and strengthening, which limits reductions of these as temperature increases to and above the transition temperature (Chap. 6, Sec. III.E). Thus some have reported both toughening and strengthening of Al2O3 with nontransforming ZrO2 additions, e.g. Langlois and Konaztowicz [35] reported (I) toughness increasing more rapidly at lower additions of cubic ZrO2 particles but increasing by 100+% at the upper addition of 30 v/o, while strength increased by 75% at 10 v/o addition and was then constant at 800 MPa.
The second point is that the processing environment can be important, as is shown by various results such as the use of HIPing. Thus Tsukuma et al. [36] showed strength maxima of 2.5 GPa at 20 w/o Al2O3 in 2Yor 3Y-TZP, which was fairly consistent with CNB, but not I, toughness–composition trends. Hori et al. [37] showed that while sintering of Al2O3 with 0–30 w/o unstabilized ZrO2 gave a narrow maximum of 650 MPa at 15 w/o; HIPing of the bodies after sintering not only increased the maximum strength to 800 MPa but also substantially broadened it to being essentially constant from at least 5 to 15 w/o. IF
554 |
Chapter 9 |
fracture toughness testing showed no difference between sintered and sinteredHIPed bodies, but it was inconsistent with the strength maximum, as were I toughness measurements, which showed toughness of HIPed bodies progressively falling below that of sintered only bodies as the ZrO2 content increased, since oxygen loss from ZrO2 can stabilize it, as is noted below. Tomaszewski [38] found similar but less extreme increases in strength maxima and its breadth (and similar trends for NB toughness) in vacuum versus air sintered bodies of similar composition. He showed that the improved properties of vacuum sintered bodies correlated with increased ZrO2 stabilization from oxygen deficiency resulting from vacuum sintering. While such environmental effects have received very limited attention, there are sound reasons why they are of probable broad importance, as is discussed further below.
2.Melt Processed ZTA Composites
The above processing and resultant microstructure of the ZTA composites is all via powder processing, which results in ZrO2 particles at grain boundaries and requires fine Al2O3 grain sizes for good strengths. However, melts of the Al2O3- ZrO2 system result in classical, well known eutectic, typically rod (Fig. 9.8), structures that have been used commercially for years for producing tough abrasives and have more recently been investigated as an alternate for producing possibly unique ZTA composites. Since both uses are based on melting to produce powder for making ZTA bodies, they are briefly summarized here; emphasizing strength results.
Commercial abrasives at or near the eutectic composition (70 v/o Al2O3-30 v/o ZrO2) are arc melted, quenched by casting thin sheets (e.g. 1–2 mm thick) and then ground into abrasive particles that are noted for their toughness in grinding wheels. Such materials, despite having no stabilizer added, have predominantly tetragonal ZrO2 [39] so long as fine microstructures are obtained and maintained. However, both the arc melting with graphite electrodes and their quenching, e.g. by pouring into graphite book molds to form thin sheets for practical crushing, result in a reduced, i.e. black, material. While detailed research has apparently not been conducted on this system, the following mechanism is suggested because the cast material has similar toughnesses to other ZTA bodies (Chap. 8, Sec. V.B) and progressive reduction of ZrO2 can progressively stabilize it [40,41]. Stabilization results because lattice defects the same as or similar to those from the addition of stabilizing agents are introduced by oxygen deficiencies from reduction. Thus reduced ZrO2 in ZTA composites may be partially stabilized, allowing transformation toughening or as noted below providing other, e.g. microcracking, toughening. Either is supported by the fact that oxidation of the melt-derived ZTA results in its turning white and developing extensive micro- and larger cracks, mainly at or near Al2O3–ZrO2 interfaces [42], which could also aid in maintaining sharp, tough abrasive edges for effective cutting.
Particle Dependence of Tensile Strength |
555 |
Various investigators have studied polycrystalline bodies made by consolidation of eutectic particles, especially Al2O3-ZrO2. Rice et al. [24,42] hot pressed commercially produced fusion cast and ground 70 v/o Al2O3-30 v/o ZrO2 into fairly dense bodies with strengths of > 500 MPa that were probably limited by residual porosity (due to limitations on fine grinding of the material due to its toughness). Fracture surfaces clearly revealed the eutectic structure and interaction of the crack with that structure (Fig. 9.8). The difficulty of grinding this material, much of the structure not being fine relative to the particle sizes desired for good densification, and the above noted cracking on oxidation, greatly reduced strengths and made this approach impractical for many applications. On the other hand, Krohn et al. [43] hot pressed melt-derived eutectic powder and obtained strengths of > 800 MPa, consistent with their high toughness (15 MPa·m1/2).
Homeny and Nick [44] conducted more detailed studies of eutectic Al2O3- ZrO2 powders made via plasma torch melting of particles and subsequent hot pressing of eutectic compositions that contained (a) 0, (b) 4.6, and (c) 9.5 m/o Y2O3. While these all gave high toughnesses (respectively 6.7, 7.6, and 7.9 MPa·m1/2), strengths were respectively 200, 750, and 650 MPa. The low strength was attributed to extensive microcracking (which was consistent with there being no Y2O3 and resultant 90% monoclinic ZrO2 content), which was cited as the source of the substantial toughness in this material. However, it was claimed that the two bodies with Y2O3 additions that had 100% tetragonal ZrO2 did not exhibit transformation toughening: toughening was attributed to crack bridging as well as substantial formation of strings of thin deformed material (much finer than grains or substantial fragments of them) bridging the cracks in the wake region.
3.Other ZrO2 Toughened Composites and Flaw Size Considerations
Briefly consider examples of results for what is probably the next most investigated ZrO2 toughened system, namely that with a mullite matrix. Data of Yuan et al. [45] showed strength increasing from 100 to 125 MPa, i.e. by up to 25%, as ZrO2 additions increased from 0 to 25 v/o (Fig. 11.2), with most of the increase occurring by 10 v/o. The levels and trend of the strength increase are consistent with that for toughness (Chap. 8, Sec. V.B) both as measured as well as with probable correction for the 2.6–8.7 residual porosity, which would raise the increase in strength from 110 to 150 MPa. However, the substantial reduction of the mullite grain size (especially of larger grains) generally accompanying increased addition of the ZrO2 was probably a factor in the strength increases, and the limited increase in ZrO2 particle size (e.g. 1 to 1.5 m) as its content was increased may have been a factor in the limitation of strength increases at higher ZrO2 contents. Strengths of 330 MPa reported by Lathabai et al. [46] for reaction processed mullite + 5 w/o Al2O3 and 31 w/o ZrO2 ( half
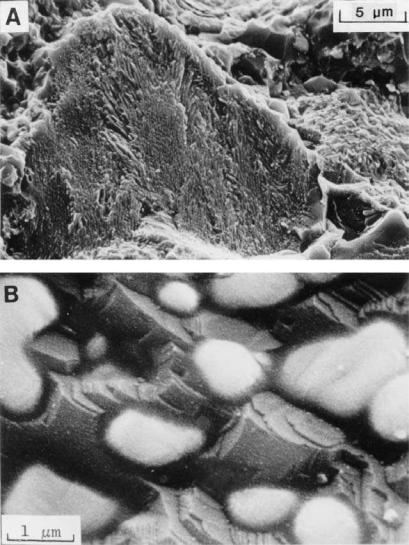
556 |
Chapter 9 |
FIGURE 9.8 Fracture surface of sample hot pressed from Al2O3-ZrO2 eutectic abrasive material. (A) shows a large fractured eutectic particle with the fracture plane parallel with the colony growth direction, and (B) shows a higher magnification of a fracture normal to the eutectic rod structure. The white phase is ZrO2 and the darker phase is Al2O3. (From Ref. 24. Published with permission of Noyes Publications.)
Particle Dependence of Tensile Strength |
557 |
tetragonal and half monoclinic) must be due in substantial part to the finer ZrO2 particle size and especially the finer mullite grain size (e.g. 1 m), since no evidence of transformation toughening was found. On the other hand, Ishitsuka et al. [47] showed strengths of TZP steadily decreasing as mullite was added, though again the value of 160 MPa for the pure mullite was probably low due to the larger G, since again the addition of ZrO2 reduced the mullite grain size. However, the only indication of the high toughness values of 12 MPa·m1/2 for 10–30 v/o ZrO2 on strengths was a slight strength decrease at 10 v/o mullite; otherwise there was a smooth continuous strength decrease with increased mullite addition in contrast to substantially greater than proportional toughness decreases from 30 to 50 and 90–100 v/o mullite.
Consider now evaluations of flaw sizes and effects. Failure causing flaws have frequently been found at fracture origins in ZTA samples showing failure from typical, mainly machining, flaws and processing defects, by various investigators. Rice [31] has investigated effects of flaw size on failure of zirconia toughened alumina conventionally produced from mixed powders. He showed that fracture toughnesses calculated from fracture strengths and observed flaw sizes and character agreed with those measured by conventional (DCB) toughness tests with larger crack sizes, but at flaw sizes < 50 m they showed progressive decreases as flaw size decreased (Fig. 9.9). This was interpreted as being due to mismatch stresses between grains of both materials increasingly contributing to the failure stress as flaw sizes became smaller, ultimately approaching the grain size, i.e. identical to monolithic ceramics, including TZP bodies (see Fig. 3.35) [32].
Finally consider the flaw sizes implied by the strengths and toughnesses, e.g. their ratio as previously discussed by Rice [24,31]. All results for additions of partly stabilized ZrO2 in his previous survey indicated reasonable flaw sizes of 30–100 m except those of Claussen [23,32], which gave 100 to > 2000m. Ono et al.’s [48] (and Becher’s [25]) addition of totally unstabilized ZrO2 also gave reasonable flaw sizes at lower, especially at 10, v/o, beyond which Ono et al.’s additions gave larger calculated flaw sizes, e.g. to > 2000 m. On the other hand, flaw size calculations from Ono et al.’s data for additions of partially stabilized ZrO2 gave reasonable flaw sizes across the complete range of additions from 0 to 100% ZrO2 [32]. Further, all data indicating reasonable flaw sizes also indicated flaw size minima at intermediate ZrO2 contents, e.g. 20 w/o, where toughening was at or near its maximum. Calculations from Ono et al.’s data also indicates a flaw size minimum at 80–90 w/o ZrO2, i.e. again indicating another toughening maximum [32]. More recently reviewed data in this and the previous chapter is generally consistent with these trends from earlier results. Thus Homeny and Nick’s data for eutectic compositions with no stabilizer indicated microcracking give lower strengths but substantial toughness,
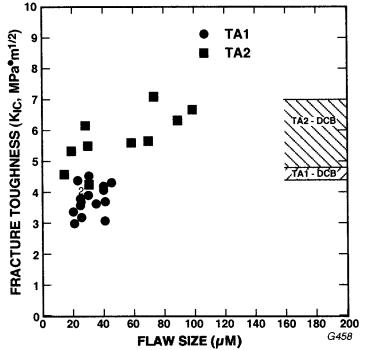
558 |
Chapter 9 |
FIGURE 9.9 Fracture toughness calculated from the applied stress at fracture and the flaw size and character at the fracture origin for commercially produced zirconia toughened alumina (Diamonite Products). Note the general agreement with conventional (DCB) toughness tests (cross-hatched areas) at large flaw sizes, but progressively lower values at smaller flaw sizes attributed to increasing contributions of microstructural stresses as for monolithic ceramics (Fig. 3.25). (From Ref. 31. Published with permission of the American Ceramic Society.)
and hence very large calculated flaw sizes, e.g. G > 1000 m [44]. Compositions with some ZrO2 stabilizer give similar toughnesses but higher strengths, and thus much lower and more reasonable calculated flaw sizes, e.g. of the order of 100 m. Similarly, data for TZP materials gives very large calculated flaw sizes at low levels of partial stabilization (where strengths are low, quite possibly due to microcracking) and reasonable flaw sizes at higher strengths and levels of stabilization. Konsztowicz and Langois [49] pursued this issue of calculated flaw sizes further for Al2O3- ZrO2 composites showing differences in calculated flaw sizes using I versus NB toughnesses and logical correlations between processing and calculated flaw sizes, and minimum flaw sizes at or near compositions for toughening maxima.
Particle Dependence of Tensile Strength |
559 |
Limited evaluations have been made of flaws in other ZrO2 toughened bodies. Evaluation of Yuan et al.’s data [45] indicates flaws of a few to several hundred microns, i.e. > normal strength controlling flaws. This is consistent with either R-curve effects increasing toughness at large (but not at normal) crack sizes, the occurrence of microcracking, or both. The low strengths of 100 MPa and thermal shock results, including some increase in toughness with decreased Young’s modulus and strength, all indicate effects of microcracking.
C.Polycrystalline Oxide Matrix Composites Without Transformation Toughening
The above ZrO2 toughened composites show that toughness and strength generally follow each other when reasonable homogeneous bodies are made within the range where transformation toughening is dominant. However, many other composites do not show such consistent behavior of toughness and strength and in fact commonly show opposite trends with basic microstructural variables, especially of the dispersed particle size. In particular it is shown that while toughness often increases as particle size increases, the opposite is true for strength, i.e. it typically increases as particle size decreases but may pass through a maximum at finer particle sizes. This section addresses behavior of polycrystalline composites without transformation, showing the noted differences and drawing upon, but also extending, recent reviews of some of these composites [24,32,50,51].
Tiegs and Bowman [51] have shown Al2O3-20% SiC particulate composite having a distinct σ maximum, i.e. increasing rapidly from 350 MPa at fine SiC particle sizes to a maximum of 530 MPa at D 2 m, then decreasing rapidly and then more slowly to 110 MPa at D 17 m. He attributed most or all of the decrease with increasing SiC particle size to microcracking, which is logical because Eq. (2.4) would predict that spontaneous cracking could start at D 1–2m. A maximum of relative strength was also shown by Yasuoka et al. [52] at D < 1 m, greater than the relative maximum of toughness but then decreasing to lower relative values than relative toughness by D 6 m for strengths. Both strength and toughness were normalized for the matrix G, since they showed that the matrix G was decreased by the SiC additions and that this added to the strength increases. This effect of the addition of SiC in reducing the matrix G was also shown by Thompson and Kristic [53], as was the benefit of fine SiC particle size. Thus they reported that the addition of 0.5 m SiC reduced the Al2O3 G from 15 m with no SiC addition to 2+ m at φ = 0.1 and < 1 m at φ= 0.2. These changes in G dominated strength increases from 290 to 490 MPa as a function of φ (maximum at φ= 0.15), with a limited decrease at φ= 0.2. Strength increased through a maximum in contrast with that of (CNB) toughness, being independent of φ at 4.2 MPa·m1/2, except for an increase of 15% at φ=0.2 (in contrast to a strength decrease there). These differing changes of strength and