
Supersymmetry. Theory, Experiment, and Cosmology
.pdf
The LSP and dark matter 109
χ |
f |
χ |
f |
|
~ |
|
A |
|
f |
|
|
χ |
– |
χ |
– |
f |
f |
||
χ |
W |
χ |
Z |
|
+ |
|
0 |
|
χ i |
|
χ i |
χ |
W |
χ |
Z |
χ |
W |
χ |
W |
|
χ+i |
|
A |
χ |
H + |
χ |
H + |
χ |
Z |
χ |
Z |
|
χ 0 |
|
Z |
|
|
|
|
|
i |
|
|
χ |
H,h |
χ |
H,h |
χ |
A |
χ |
A |
|
χ 0 |
|
Z |
|
|
|
|
|
i |
|
|
χ |
H,h |
χ |
H,h |
χ |
f |
|
Z |
χ |
f |
|
χ |
Z |
|
A |
χ |
H,h |
χ |
A |
|
A |
χ |
H,h |
Fig. 5.1 Main annihilation channels of the lightest neutralino χ at rest.
5.5.4LSP interaction with matter
It is important to be able to determine as precisely as possible the interaction of the LSP neutralino with nonrelativistic matter in order to make predictions for its direct detection as well as its capture by material bodies.
Since we are considering matter in the nonrelativistic limit, we first recall that
ϕ
0
¯ ¯ µ †
is a two-component spinor field. Then, ΨΨ and Ψγ Ψ yield the scalar ϕ ϕ whereas
11We use here the Dirac representation for gamma matrices: γ0 |
= β = |
1 |
0 |
, γi = βαi with |
||||||
0 |
−1 |
|||||||||
αi = |
0 |
σi |
and γ5 |
= |
0 1 |
. For example, Ψ¯ γ0Ψ = Ψ† Ψ = ϕ† ϕ whereas Ψ¯ γiΨ = Ψ† αiΨ = 0. |
||||
σi |
0 |
1 0 |

110 The minimal supersymmetric model
¯ ¯ ¯
Ψγµγ5Ψ and Ψσµν Ψ yield the vector ϕ†σiϕ and Ψγ5Ψ vanishes in the nonrelativistic limit.
Because of the Majorana nature of the neutralino (which implies χγ¯ µχ = 0 and χσ¯ µν χ = 0, see (B.40) of Appendix B), the most general interaction is described at
the level of quarks, by the e ective four-fermion Lagrangian: |
|
|
L = i |
di χγ¯ µγ5χ q¯iγµγ5qi + fi χχ¯ q¯iqi . |
(5.83) |
One is thus left with the axial-vector (also called spin dependent) interaction with coefficient di and the scalar interaction (also called spin independent) with coe cient fi.
From the interactions derived in Section 5.5.2, we see that (cf. Fig. 5.2) scalar (resp. spin-dependent) interactions arise through the exchange of H, h (resp. Z) in the t channel. The exchange of a sfermion in the s or t channels leads through Fierz reordering to both types of interactions.
It turns out that the scalar contribution is dynamically reduced with respect to the spin-dependent one. As we will see in the next section, this is compensated experimentally by the fact that it behaves coherently and the e ects of all the nucleons in a nucleus add up. For the time being, we will identify the origin of the dynamical reduction at the level of a single nucleon.
We first consider squark exchange. A large contribution of this type would require the lighter squarks of the third family and thus the implementation of sfermion mixing. More precisely, the contributions to the couplings di and fi in (5.83) read
|
1 |
|
2 |
a2 |
+ b2 |
|
|
||
di|q˜ exch. = 4 |
r |
− |
ir |
ir |
, |
|
|||
|
|
|
|
|
|||||
|
m˜ q2ir |
|
|
(mχ + mqi )2 |
|
||||
|
|
=1 |
|
|
|
|
|
|
|
f |
= 1 |
2 |
|
|
air2 − bir2 |
|
. |
||
i|q˜ exch. |
− 4 |
r |
|
|
− |
(mχ + mqi )2 |
|
||
m˜ q2ir |
|
|
|
||||||
|
|
|
=1 |
|
|
|
|
|
|
(a) |
|
|
|
|
(b) |
|
|
|
|
χ |
|
|
χ |
|
|
|
χ |
|
|
|
|
|
|
|
|
|
|
|
|
H,h |
|
|
|
|
|
|
|
q |
|
|
|
|
|
|
|
|
|
|
|
q |
|
|
q |
|
|
|
q |
|
|
(c) |
|
|
|
|
(d) |
|
|
|
|
χ |
|
|
q |
|
|
|
χ |
|
|
|
|
|
|
|
|
|
|
|
Z |
q |
|
|
|
|
|
|
|
|
|
q |
|
|
χ |
|
|
|
q |
|
|
(5.84)
(5.85)
χ
q
χ
q
Fig. 5.2 Scalar (a), (b), (c) and spin-dependent (b), (c), (d) interactions of the lightest neutralino χ with matter.
The LSP and dark matter 111
The couplings air and bir describe scalar and pseudoscalar LSP–squark–quark interactions:
2 |
air + birγ5 χq˜ir + h.c., |
|
Lχqq˜ = q¯ir |
(5.86) |
i r=1
where the index r refers to the two mass eigenstates in the case of sfermion mixing (see (5.58)). They can be obtained from (5.81) and read explicitly
ai1 = − 12 cos θqi (Xi + Ziq) + sin θqi (Yi + Ziq ) ,
bi1 = − 21 cos θqi (Xi − Ziq) + sin θqi (Yi − Ziq ) , |
(5.87) |
with cos θqi replaced by − sin θqi and sin θqi replaced by cos θqi for r = 2.
Realizing that |air | = |bir | in the chiral limit (Ziq goes to zero with the fermion mass), we see that fi vanishes in this limit. This is true also for the contribution coming from H, h exchange since their couplings to quarks are proportional to the mass, see Table 5.3. The scalar contribution is thus naturally disfavored for the light quarks that are abundant in nucleons. Heavy quarks couple to nucleons only through a loop [123, 124]. The combined e ects lead to a dynamical suppression of the scalar interaction. We may note that, going to large values of tan β may significantly increase the cross-section because, as can be seen from Table 5.3, it enhances the couplings of H, h to down-type quarks [123].
Regarding the spin-dependent cross-section, we infer from (5.77) that a large contribution from Z exchange requires a large Higgsino component, which is disfavored if we want a substantial relic abundance.
To give an order of magnitude, in models where the LSP is mostly a bino, one typically obtains spin-dependent cross-sections σχSD−p in the 10−7 to 10−5 pb and scalar cross-sections σχSI−p in the range 10−10 to 10−7 pb range.
5.5.5Direct detection
Goodman and Witten (1985) were the first to propose to use bolometers to detect dark matter candidates. Typically, the event rate R is given by the number NN = NA/A of nuclei in the target (NA is Avogadro’s number and A the atomic number) times the flux F = ρχvχ/mχ (ρχ is the local WIMP density and vχ the average WIMP velocity) times the cross-section σ. Putting typical numbers, this gives
|
1 |
|
ρχ |
vχ |
|
100 GeV |
|
σ |
|||||
R = 4.7 evts.kg−1.day−1 |
|
|
|
|
|
! |
|
|
|
|
|
|
. |
A |
0.3GeV/cm3 |
300km/s |
mχ |
1 pb |
|||||||||
|
|
|
|
|
|
|
|
|
|
|
|
(5.88) |
|
If the invariant amplitude M is a constant at low energy, the cross-section reads |
|||||||||||||
|
|
µ2 |
|M|2 |
|
|
|
|
|
(5.89) |
||||
|
|
|
σ = |
|
|
|
|
|
|
||||
|
|
|
π |
|
|
|
|
|
where µ is the reduced mass (µ−1 = MA−1 + m−χ 1). For the processes discussed in the preceding section, M is typically of the form g2/M 2, where g is a gauge coupling and
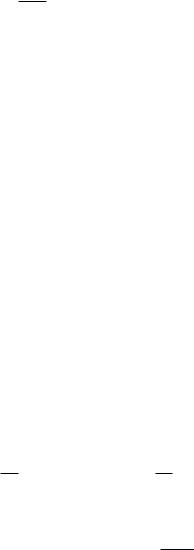
112 The minimal supersymmetric model
M a heavy scale (Z mass, Higgs mass or squark mass) which is larger than say 100 GeV. This gives, in the most optimistic case, a cross-section of the order of a picobarn, and thus rates of a few events per kg and per day. This is typically the rate obtained by present day experiments, which thus start to put some limits on the supersymmetric parameter space. This is also the rate of radioactive or cosmic ray background, which urges the need to perform these experiments in underground laboratories.
For a target nucleus of mass MA A GeV, the momentum transferred is |q2| = 2µ2vχ2 (1 − cos θ ) where θ is the scattering angle in the center of mass frame. The recoil energy is then
Q |
≡ |
|q2| |
= |
|
mχ2 MA |
v2 |
(1 |
− |
cos θ ). |
||||
|
(mχ + MA)2 |
||||||||||||
|
2MA |
χ |
|
|
|||||||||
For example, if mχ MA, |
|
|
|
|
|
|
|
|
|
|
|
||
|
|
|
|
mχ |
2 |
vχ |
|
2 |
1 |
|
|||
Q |
|
|
|
|
|
|
|
keV. |
|||||
1 |
GeV |
300 km/s |
|
A |
(5.90)
(5.91)
Hence, one expects an energy deposited in the detector in the range of a few keV. We now discuss separately the spin-dependent and spin-independent contributions
to the cross-section. As alluded to above, the scalar interaction adds coherently among the nucleons: this means that the invariant amplitude is proportional to A and the cross-section (5.89) scales like µ2A2. This represents a large enhancement factor if one uses heavy nuclei. Typical examples are germanium (Z = 32, A = 76) or xenon (Z = 54, A = 136).
More precisely the scalar di erential cross-section reads |
|
||||
|
dσSI |
= |
1 |
[Zfp + (A − Z)fn]2 F 2(Q), |
(5.92) |
|
d q 2 |
πv2 |
|||
| | |
|
χ |
|
|
where fp and fn are the e ective neutralino couplings to proton and neutron respectively [18] and F (Q) is the nuclear form factor.
For the spin-dependent contribution, ones introduces the nucleonic matrix element
N |q¯iγµγ5qi|N ≡ 2sµ∆qi(N) |
(5.93) |
where sµ is the spin vector of the nucleon N . The experimental values of the constants
thus introduced are ∆u(p) = ∆d(n) = 0.78, ∆d(p) = ∆u(n) |
= |
− |
0.5 and ∆s(p) = |
|||||||||||||||||||
∆s(n) = −0.16. Then, the relevant part of (5.83) reads |
|
|
|
|
|
|||||||||||||||||
LSD = 2√ |
|
|
|
χγ¯ µγ5χ [apps¯ µp + anns¯ µn] , |
|
|
|
|
|
|||||||||||||
2 |
|
|
|
|
|
|||||||||||||||||
1 |
|
|
i= |
|
(p) |
|
1 |
|
|
(n) |
|
|
||||||||||
ap = |
√ |
|
|
u,d,s di∆qi |
|
, |
an = |
√ |
|
i=u,d,s di∆qi |
|
. |
(5.94) |
|||||||||
2 |
|
2 |
|
|||||||||||||||||||
Then the spin-dependent di erential cross-section reads |
|
|
|
|
|
|||||||||||||||||
|
|
|
|
|
dσSD |
= |
|
8 |
λ2J(J + 1) |
S(|q|) |
, |
|
|
|
(5.95) |
|||||||
|
|
|
|
|
|
πvχ2 |
S(0) |
|
|
|
||||||||||||
|
|
|
|
|
d|q|2 |
|
|
|
|
|
|
|
|
|
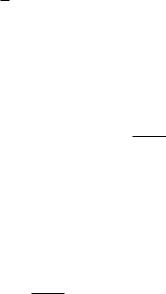
The LSP and dark matter 113
where S(|q|)/S(0) is the nuclear spin form factor, J is the total spin of the nucleus, and λ is a nucleonic matrix element that basically describes the fraction of the total spin that is due to the spin of the nucleons:
λ = |
1 |
[ap Sp + an Sn ] , |
(5.96) |
J |
where Sp,n is the expectation value of the proton (neutron) group spin content of the nucleus.
To recapitulate, the di erential detection rate is calculated to be
|
dR |
|
4 ρχ |
|
|
|
|
|
|
|
|
2 |
|
|
2 |
|
|
|
|||||
|
|
|
= |
|
|
|
T (Q) [Zfp + (A − Z)fn] |
|
F |
|
(Q) |
|
|
||||||||||
|
dQ |
π3/2 |
mχvχ |
|
|
|
|
||||||||||||||||
|
|
|
|
|
|
|
|
|
|
|
+8λ2J(J + 1) |
S(|q|) |
|
|
, |
(5.97) |
|||||||
|
|
|
|
|
|
|
|
|
|
|
S(0) |
|
|
||||||||||
|
|
|
|
|
|
|
|
|
|
|
|
|
|
|
|
|
|
|
|||||
where |
|
|
|
|
|
√ |
|
|
∞ f |
(v) |
|
|
|
|
|
|
|
|
|
||||
|
|
|
|
|
|
|
|
πv |
|
|
|
|
|
|
|
|
|
||||||
|
|
|
|
|
T (Q) ≡ |
|
χ |
vmin |
|
χ |
|
dv |
|
|
|
|
|
(5.98) |
|||||
|
|
|
|
|
|
2 |
|
v |
|
|
|
|
|
||||||||||
integrates over the neutralino velocity distribution fχ(v) (vmin = µ−1 |
|
|
|||||||||||||||||||||
MAQ/2). |
For the sake of illustration, let us take a simple Maxwellian distribution of velocities |
||||||||||||
in the halo (isothermal model): |
|
|
|
|
|
|
|
|
|
|
|
" |
|
|
|
|
|
|
|
|
|
|
|
|
|
fχ(v) = |
4v2 |
2 |
2 |
|
|
(5.99) |
||||||
v3 |
√ |
|
e−v |
/vχ . |
|
|
||||||
π |
|
|
||||||||||
|
|
|
χ |
|
|
|
|
|
|
|
|
|
Then (5.98) gives simply |
|
|
|
|
|
|
|
|
|
|
|
|
|
|
v2 |
|
|
|
|
|
|
|
QMA |
|
|
T (Q) = exp |
|
min |
|
|
|
= exp |
|
. |
(5.100) |
|||
− vχ2 |
|
|
2µ2vχ2 |
|||||||||
|
|
|
|
|
− |
|
|
The total rate R is, in this case, easily computed. Assuming an experimental cut-o ET for the recoil energy measured,
∞ dR |
|
ρχ |
− |
ET MA |
|
|
||
R = ET |
|
dQ |
|
exp |
|
, |
(5.101) |
|
dQ |
mχ |
2µ2vχ2 |
where we have made explicit in the last term the dependence in the neutralino density and mass. If an experiment puts an upper limit on R, this translates into a upper limit on ρχ of the order of
|
ET |
|
MA |
2 |
|
mχ exp |
1 + |
. |
|||
2MAvχ2 |
mχ |
This behaves linearly with mχ for large mχ and grows exponentially for small mχ. Thus, exclusion plots typically look like Fig. 5.3: assuming a given value of ρχ leads to the exclusion of neutralino masses in a range [mminχ , mmaxχ ].
Since the rate depends on the velocity of the incident WIMP, one expects an annual modulation due to the motion of the Earth in the Galactic frame. More precisely, the
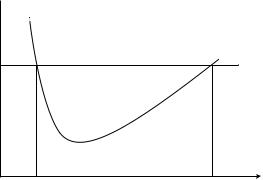
114 The minimal supersymmetric model
ρχ
Local galactic density of halo
Excluded
m |
min |
m |
max |
mχ |
χ |
|
χ |
|
|
Fig. 5.3 Typical form of the exclusion plot for a WIMP in the plane local density versus mass.
Earth motion is a superposition of its rotation around the Sun and of the rotation of the Sun around the Galactic Center (with velocity v = 220 km/s):
v = v [1.05 + 0.07 cos (2π(t − tp)/year)] , |
(5.102) |
where t is measured in days and tp 153 days (June 2) is the day when the direction of the Earth’s motion around the Sun matches the direction of the Sun’s motion around the Galactic Center [164]. The numerical coe cients reflect the 60◦ angle between the two planes of rotation. The yearly modulation comes from the fact that, in the lab (Earth) frame, one must shift the velocity distribution: f (v + v ).
5.5.6Indirect detection
WIMPs annihilate and their annihilation products can be detected. The variety of these particles (gamma rays, neutrinos, positrons, antiprotons, antinuclei) leads to expect to extract rich information from correlated observations. Because the annihilation rate is proportional to the square of dark matter density, one increases the expected flux by looking at places where the dark matter accumulates. Typical places are the center of the Earth or the Sun: WIMPs loose energy by scattering with the nucleons of matter and sink to the center. Or the Galactic Center: the density profile of the dark matter halo is expected to grow as a power law (ρ(r) r−γ ); this e ect may even be enhanced by adiabatic accretion on the central massive black hole leading to a central “spike” [201].
WIMPs which have accumulated in the Earth or the Sun annihilate into ordinary particles. Most of the decay products of these particles will be absorbed, except for high energy neutrinos (with typical energy 13 to 12 the WIMP mass) which pass through the Earth or the Sun. Thus energetic neutrinos are a signature for dark matter. Interactions of such neutrinos in the Earth give energetic muons which are detected in large underwater (or ice) detectors such as AMANDA, ANTARES, or NESTOR.
The capture rate of neutralinos is proportional to the nucleus–neutralino elastic
SI,SD
scattering cross-section, and thus depends on σχ−p,n. In the case of the Earth, scalar

The LSP and dark matter 115
interactions are favored because of the zero spin of Fe56. For the Sun, the spin of hydrogen allows spin-dependent interactions and capture is more e cient.
On general grounds, the annihilation cross-section of neutralinos at rest (a good approximation in the case of neutralinos trapped in the center of a celestial body) into massless fermions vanishes [195]. Indeed, Fermi statistics imposes that the spin of the two neutralinos in the initial state (S-wave) are opposite. In the final state, the required spin flip is ensured by a mass insertion; hence the cross-section is proportional to the
mass of the fermions. Direct annihilation into neutrinos is thus strongly disfavored and
→ ¯ ¯ + − + − → neutrinos are produced as secondary particles: χχ tt, bb, cc,¯ τ τ , W W , ZZ
Xν. The more energetic the neutrinos are, the better their conversion into muons and the longer the distance they cover. Hence energetic neutrinos are more easy to detect.
Annihilation of neutralinos in the halo also gives monoenergetic photons (through the one-loop processes χχ → γγ, γZ) but also a continuous spectrum of photons through the decay of annihilation products (mostly from the decay of π0 produced in hadronization). Generally speaking, the annihilation flux is proportional to (i) the number of annihilations per second in a unit volume σannv ρ2(r)/M 2 for dark matter particles of mass M and annihilation cross-section σann, (ii) the spectrum dNi/dE of secondary particles of type i. Performing an integration over the line of sight, one obtains the observed flux of secondary particles of type i in a direction making an angle ψ with the direction of the galactic center:
σannv dNi |
line of sight ds ρ2(r) |
|
Φi(E, ψ) = 4πM 2 dE |
(5.103) |
where the galactocentric coordinate is expressed in terms of the coordinate s along the line of sight and the angle ψ as: r2 = s2 + R02 − 2sR0 cos ψ (R0 8 kpc is the solar distance from the galactic center).
There is thus a strong dependence on the density profile of dark matter ρ(r) which is poorly known. It is usually parametrized as
ρ(r) = |
ρ0 |
, |
(5.104) |
(r/R)γ [1 + (r/R)α](β−γ)/α |
where R is a characteristic length and α, β, and γ are parameters. Table 5.5 gives their values obtained in typical models based on N -body simulations. Because of the strong model dependence of the astrophysics input in the computation of the
¯
flux, it proves useful to factorize it and to introduce J(∆Ω), the average of the function
J(ψ) = line of sight |
ds |
|
ρ(r) |
! |
2 |
|
(5.105) |
||||
|
|
|
|||
8.5 kpc |
0.3 GeV/cm3 |

116 The minimal supersymmetric model
Table 5.5 Values of the parameters used to parametrize dark matter profiles for typical models: Navarro–Frenk–White (NFW) [293], Moore et al. [289] and isothermal [31].
Model |
α |
β |
γ |
R (kpc) |
J¯(10−3) |
NFW |
1.0 |
3.0 |
1.0 |
20 |
1.35 × 103 |
Moore |
1.5 |
3.0 |
1.5 |
28 |
1.54 × 105 |
Isothermal |
2.0 |
2.0 |
0 |
3.5 |
2.87 × 101 |
¯ −3
over a region of solid angle ∆Ω centered on ψ = 0. The values of J(10 ) are shown in Table 5.5 for various models: they show that the prediction for the astrophysical factor based on N -body simulations varies by several orders of magnitude!
Then the flux coming from a solid angle ∆Ω reads
Φi(E, ∆Ω) = 5.6 × 10−12 |
dN |
|
|
σannv |
|
|
1 TeV |
i |
|
|
|||||
dE |
|
1 pb |
|
M |
2
¯ −2 −1
J(∆Ω)∆Ω cm s .
(5.106)
The typical energy of gamma rays from neutralino annihilations is in the GeV–TeV range. Since the interaction length of photons with matter is of the order of 30 g cm−2 (to be compared with the thickness of the atmosphere 1030 g cm−2), only space missions like GLAST, which follows EGRET launched in 1991, will be able to provide a significant test for supersymmetric models. However ground Cherenkov telescopes such as H.E.S.S, MAGIC, and VERITAS, which measure the Cherenkov light emitted by the particles produced in the cosmic gamma ray shower, show some sensitivity to models with a large neutralino mass.
The annihilation of neutralinos can also produce positrons. Moreover, the secondary electrons and positrons propagate in the galactic magnetic field, producing synchrotron radiation. Typical frequencies are of the order of a few hundred MHz. The corresponding observations provide significant constraints on the positron flux expected. Other annihilation products include antiprotons and antideuterons. Given the uncertainties on the astrophysical quantities, the level at which these particles are expected in a given supersymmetric model is di cult to predict. On the other hand, ratios of fluxes corresponding to di erent species are less sensitive to the details of halo models.
5.5.7Dark matter codes
Public codes exist which provide, for a given supersymmetric model, relic abundances, as well as cross-sections, expected fluxes, etc. We refer the interested reader to the webpage of two of these codes:
DARKSUSY [200]: http://www.physto.se/edsjo/darksusy/ MicrOMEGAs [29]: http://wwwlapp.in2p3.fr/lapth/micromegas
Nonminimal models 117
5.6Nonminimal models
We end this chapter with a discussion of the simplest extension of the MSSM, obtained by adding a gauge singlet S and all its renormalizable interaction [129,295]. This model is called the next-to-minimal supersymmetric model (NMSSM, sometimes also referred to as the (M+1)SSM). We will see in Chapters 9 and 10 that singlets naturally appear in the context of grand unified models or string models.
The most general superpotential compatible with the symmetries is:
W = 21 µS S2 + 61 κS S3 + λS SH2 · H1 + W (2) + W (3), |
(5.107) |
where W (2) and W (3) are the standard MSSM quadratic and cubic terms given in equations (5.1) and (5.2).
One of the motivations for the NMSSM is that it provides a framework to account for a dynamical origin of the µ term. Indeed, let us assume the existence of a discrete symmetry that prohibits any quadratic term in the superpotential: µ = µS = 0. A rationale behind this assumption is that the typical mass scale of the fundamental theory underlying this e ective low energy theory is very large: any dimensionful parameter should be fixed by this superheavy scale or vanishing. The low energy theory then has only dimensionless parameters such as κS , λS and the Yukawa couplings, and its mass scale appears only through symmetry breaking. Indeed, if S acquires a vacuum expectation value, then we obtain an e ective µ-term with
|
µ = λS S . |
(5.108) |
Correspondingly, soft terms read |
|
|
VSB|NMSSM = VSB|MSSM + |
61 AκκS S3 + AλλS SH2 · H1 + h.c. , |
(5.109) |
which generates an e ective Bµ term: Bµ = AλλS S .
The spectrum of the NMSSM is the one of the MSSM plus one scalar, one pseudoscalar, and one Weyl fermion (often referred to as the singlino). The scalar mixes with h0 and H0, the pseudoscalar with A0. The singlino mixes with the other neutralinos: the neutralino mass matrix Mn is now five-dimensional. In most of the parameter space, these mixings are small and the fields associated with the S superfield basically decouple. Otherwise, one may encounter large di erences with the phenomenology of the MSSM [135].
Further reading
•H. Haber and G. Kane, The search for supersymmetry: probing physics beyond the Standard Model, Physics Reports 117 (1985) 75.
•G. Jungman, M. Kamionkowski and K. Griest, Supersymmetric dark matter, Physics Reports 267 (1996) 195–373.

118 The minimal supersymmetric model
Exercises
Exercise 1 Explain in detail why the MSSM has 105 real parameters besides the 19 of the Standard Model, i.e. 124 real parameters in total.
Hints: See Chapter 12, Section 12.1.
Exercise 2 Show that the potential V ≡ VF + VD + VSB given by (5.10)-(5.12) has only charge-conserving minima: H1± = 0 = H2± .
Exercise 3 Compute the mass matrices corresponding to the scalar potential of the MSSM (Section 5.3.1) for the neutral scalars (Re Hi0) and pseudoscalars (Im Hi0). Deduce (5.28)–(5.30) and (5.32)–(5.33).
Hints: |
m12 |
|
|
|
|
|
|
|
|
|
|
|
|
|
|
|
|
|
|
|
|
|
|
|
|
|
|
g2 |
+ g 2 |
|
|
|
|
|
|
g2 |
+ g 2 |
|
|
||||||||||
|
+ |
|
|
|
|
(3v12 − v22) |
Bµ − |
|
|
|
|
|
v1v2 |
|||||||||||
Msc2 = |
− |
4 |
2 |
4 |
2 |
|
||||||||||||||||||
|
|
|
|
|
|
|
|
|
|
|
|
|
|
|
|
|
|
− |
|
|||||
|
|
|
|
|
|
|
g2 + g 2 |
|
|
|
|
|
g2 + g 2 |
|
|
|
|
|||||||
|
|
|
Bµ |
|
|
v1v2 |
m22 + |
(3v22 |
|
v21) |
||||||||||||||
|
|
|
|
|
|
|
|
|
||||||||||||||||
Mps2 = |
m2 |
+ |
g2 + g 2 |
(v2 |
|
v2) |
|
|
|
|
B |
|
|
|
. |
|||||||||
|
|
|
|
|
|
|
|
|
||||||||||||||||
1 |
|
|
|
4 |
1 |
− |
2 |
|
|
|
− |
µ |
|
|
|
|
||||||||
|
|
|
|
|
|
|
|
|
|
|
|
|
|
|
|
− |
||||||||
|
|
|
|
|
|
|
− |
|
|
|
|
|
|
|
|
|
4 |
|
|
|
|
|||
|
|
|
|
|
|
|
|
|
|
|
|
|
|
|
g2 + g 2 |
|
|
|
|
|
||||
|
|
|
|
|
|
|
|
Bµ |
|
|
m22 + |
|
|
(v22 v21) |
Exercise 4 Using the Lagrangians presented in Chapter 3, prove (5.43)–(5.44) where
λ |
± ≡ |
(λ1 |
|
|
|
µ ≡ |
µ |
µ |
|
|
|
|
λ2)/√2 is the supersymmetric partner of W ± |
(A1 |
A2 )/√2. |
Hints: Use equations (3.30) and (3.52) of Chapter 3. Note that, because λ1 and λ2 are
Majorana spinors and because charge conjugation implies hermitian conjugation, one
√
has λc± = λ . Also λ± = (λ1 ± iλ2)/ 2.
Exercise 5 Compute the spectrum of charginos and neutralinos in the limit case where
MW , MZ M1 < M2 < |µ|.
Hints: To leading order in M 2 |
/µ, |
|
|
|
|
|
|
|
|
|
|
|
|||||||||
|
|
|
|
|
|
|
|
W,Z |
|
|
|
|
|
|
|
|
|
|
|
||
m |
|
= M |
2 − |
M 2 |
|
M2 + µ sin 2β |
|
, m |
|
|
= µ + M 2 |
|µ| + M2sgn(µ) sin 2β |
, |
|
|||||||
|
|
µ2 − M22 |
|
|
|
|
|
|
|||||||||||||
|
χ1± |
|
W |
|
|
χ2± |
| | |
W |
µ2 − M22 |
|
|
|
|
||||||||
mχ10 |
= M1 − MZ2 sin2 |
θW |
M1 + µ sin 2β |
, mχ20 |
= M2 − MZ2 cos2 θW |
M2 + µ sin 2β |
, |
||||||||||||||
|
µ2 − M12 |
|
µ2 − M22 |
||||||||||||||||||
|
0 |
|
|
|
2 |
|
|
|
|
|
|
|
µ ± M1 cos2 θW ± M2 sin2 |
θW |
|
|
|||||
|
|
|µ| |
+ MZ (1 |
sgn(µ) sin 2β) | | 2(|µ| ± M1)(|µ| ± M2) |
. (5.110) |
||||||||||||||||
mχ3,4 = |
Exercise 6 Check the terms in the d-type squark mass matrix (5.54) or slepton mass matrix (5.57).
Exercise 7 We wish to study the evolution with time of the number N of neutralinos in the center of a celestial body under the influence of capture and annihilation.