
Invitation to a Contemporary Physics (2004)
.pdf
8.4. White Dwarfs |
295 |
position on the main sequence. The subsequent collapse of the core releases enough energy to heat up the layers around the core, igniting a shell of hydrogen. The luminosity generated by the shell source pushes layers lying just above, causing them to expand. The bloated envelope, fed in radiation by convective currents, gives the star the appearance of a red giant.
In a low-mass star, the next cycle of fusion involving helium burning is accompanied by a fast and copious production of energy, which might force the swollen star to shed its hydrogen-rich envelope and give birth to a planetary nebula together with a remnant core. This exposed hot core goes on to become a white dwarf.
High-mass stars evolve much more quickly, because they radiate at much higher rates, yet can manage to adapt themselves to the rapid internal changes. They evolve smoothly, undergoing contraction, raising the temperature to the level needed to ignite the ash of the previous fusion cycle, and starting a new cycle. But when iron is finally formed at the center, the nuclear energy source dries up, and the core has no recourse but to contract. The supernova explosion that ensues leaves behind a compressed neutron-rich core, which evolves into a neutron star or a black hole.
8.4 White Dwarfs
The story of the evolution of stars is the story of a death foretold: pressed by the relentless pull of gravity and surrounded on all sides by a cold and dark space, stars are fighting a battle they cannot win. Once drained of their energies, they will inevitably lose their struggle and die, either in a destructive explosion in which their ashes turn to dust, or in a lingering death in which, as a white dwarf, a neutron star or a black hole, they finally come to terms with both gravity and the universe.
8.4.1Observations of White Dwarfs
In 1844 astronomer Friedrich Bessel noticed, while examining astronomical records over many years, that Sirius, the brightest star in the northern sky, showed a wobbly motion. He interpreted the irregular motion of Sirius as being caused by the pull of an unseen massive star that gravitated around it. This invisible companion star turned out to be the first white dwarf star ever discovered, now known as Sirius B. Although it is the brightest white dwarf detected in our Galaxy, its faint glow is overwhelmed by the light of Sirius, a star ten thousand times more luminous. Just as for many other stars, the radius of Sirius B is deduced from its light spectrum. From spectral analysis, astronomers first obtain its surface temperature (26 000 K), and from its apparent brightness and an independent measurement of its distance from earth (8.6 ly), they next find its true luminosity. With the temperature and luminosity known, the star radius is derived from the familiar radiation law.
White dwarfs are common. Those that have been identified locally range in radii from 0.01 to 0.02 R and in temperatures from 5 000 to 50 000 K. In the

296 |
Bright Stars and Black Holes |
H–R diagram, they occupy a narrow band below the main sequence. The first few discovered and still the easiest to discover have temperatures near 10 000 K, and so look ‘white,’ but there is no particular temperature associated with these stars. Blue white dwarfs are rare because they cool quickly, and red ones are dim and hard to detect. But if we look hard, we will find them: the Hubble Space Telescope in the last years has found white dwarfs 30 times hotter than the sun (at 2 ×105 K) and many faint and cool ones (the brightest no more luminous than a 100 W light bulb seen at the moon’s distance).
Their masses are very di cult to determine except when they belong to visual binaries. In these cases, the Newton gravitational law, applied to the observed orbital motions, can give stellar masses, and radii are derived from knowledge of e ective temperatures and distances. The recent Hipparcos space astrometry mission, dedicated to precise parallax measurements, yields data from which masses and radii can be estimated even more reliably than before. For the four best known such stars — Sirius B, Procyon B, 40 Eri B and Stein 2051 B — with respective radii of 5846, 6680, 9465 and 7725 km, their masses have been established at 1.0, 0.60, 0.50 and 0.48 solar masses, respectively. White dwarfs therefore have average mass densities of some 1 × 109 kg/m3, one million times greater than the density of the sun (1.4 × 103 kg/m3) or the earth (5.4 × 103 kg/m3).
8.4.2Electron Degeneracy Pressure
A mass density of 1 × 109 kg/m3 implies a number density for electrons of ne = 1036/m3 (for comparison, ne = 1027/m3 in metals). This tells us that the electrons of the white dwarf’s atoms evolve considerably closer to one another here than they do in ordinary atoms but are still farther apart than the mean nucleon–nucleon distance. So they are e ectively squeezed together outside the atomic nuclei, which remain intact but stripped of their electrons. White dwarfs are composed of a dilute ion gas co-existing with a very dense electron gas.
For a gas that dense — when the distance between two nearest neighbors becomes comparable to or smaller than their de Broglie’s wavelength — quantummechanical e ects become important.14 This means, in particular, that the electrons must obey the Pauli exclusion principle: no two electrons can occupy the same single-particle quantum state. In a normal gas (e.g., metals), this is not a problem since there are not enough electrons around to completely fill up all the energy levels. But in a white dwarf, all the electrons are forced close together, and soon all the available energy levels are occupied, from bottom to top, two electrons in each. We say that the white dwarf has become degenerate. Once a star is degenerate, gravity cannot compress it anymore, because there is no more available space to be taken up — electrons cannot go where quantum mechanics forbids.
In a degenerate gas, the mean separation ∆x between an electron and its nearest neighbor is very small, and their momenta must di er by at least ∆px = h/∆x (by
14See Appendix C.

8.4. White Dwarfs |
297 |
Heisenberg’s uncertainty relation), otherwise they would violate the Pauli exclusion principle. As ∆x is small, ∆px is correspondingly large; so electrons squeezed to high densities must have high random speeds. These large random motions, which are of quantum-mechanical, not thermal, origins, give rise to a degeneracy pressure that depends only on the electron density, not on the temperature. This is because when the electrons fill up all the energy levels up to a certain maximum, the energy of the last occupied level (the Fermi energy) is well defined and depends only on the number density. So all energy-related quantities must also have that property. It turns out that the electron degeneracy pressure goes as P n5e/3 when the electrons are non-relativistic, and P n4e/3 when they are ultra-relativistic. (Such relations between pressure and density are known as ‘equations of state.’) It is important to note that the proportionality factors in both cases are completely defined in terms of fundamental physical constants. The reason that the relativistic pressure increases less quickly with increasing density than the non-relativistic pressure is that, as ∆x becomes smaller, ∆px becomes larger and, at some point, the increase of the velocity vx must saturate, because vx approaches the speed of light for very large momenta px.
Thus, in high densities, the degeneracy pressure dominates over the thermal pressure (which varies only linearly with ne) and can become strong enough to support a white dwarf against its self-gravity. Degenerate stars survive, not by internal combustion, but by the quantum-mechanical degeneracy pressure.
8.4.3Mass and Size
A white dwarf can hold up as a stable structure because the outward pressure due to its electron degeneracy, Pe n5e/3, can grow powerful enough to balance out the inward pressure arising from its self-gravity, Pg M2R−4 (where M and R stand for the star’s mass and radius). Since ne MR−3, we see that white dwarfs must satisfy a mass–radius relation15:
M = constant × R−3 .
It is a startling result: the more massive a white dwarf is, the smaller it is. In our ordinary experience, we expect, for instance, a marble twice as massive as another should have twice the volume. Not so with white dwarfs. A white dwarf twice as massive as another occupies only half the volume, because it squeezes four times more electrons together to maintain enough outward pressure to support the extra mass. Thus, an equilibrium state always exists for any reasonable mass.
But there is a limit to how much matter a white dwarf can hold. As its mass increases well above 1 M , its central density increases sharply, so that more electrons become relativistic. When all the electrons become ultra-relativistic, all reaching the speed of light, the degeneracy pressure will also attain its relativistic limit,
15The ‘constant’ actually is 2.2×10−6 M R3 (2Ye)5, where Ye is the ratio of the electron number to the nucleon number. Numerically, it is 1.5 × 1051 m3 kg for Ye = 0.5.
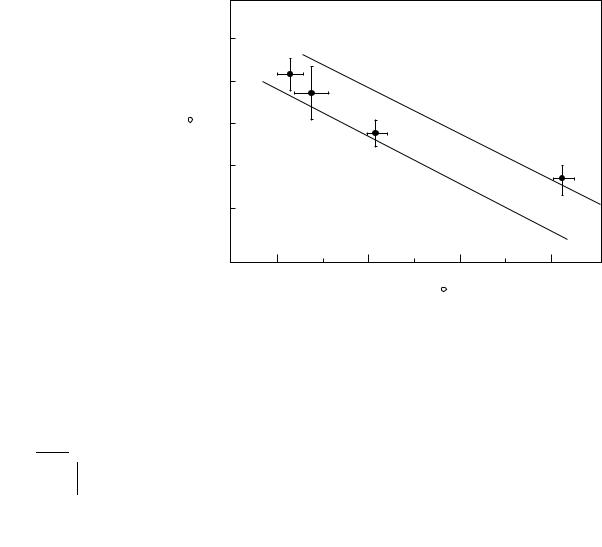
298 |
Bright Stars and Black Holes |
|
Pe |
ne4/3, or equivalently Pe M4/3R−4 |
. Since the gravitational and internal |
energies depend on R in the same way, R−4 |
, and hence go up or down in the same |
ratio, the star cannot seek stability by collapsing to a higher density. On the other hand, as the mass of the star is increased, its gravitational pressure increases as M2, whereas its internal pressure goes up more slowly as M4/3. So, there must be an upper limit to the mass of stable degenerate stars, which is the Chandrasekhar mass limit:
MCh = 1.4 (2Ye)2 M ,
so called in honor of its discoverer, Subrahmanyan Chandrasekhar (winner of the Nobel Prize for Physics in 1983). This equation, one of the most important in astrophysics, is remarkable in its simplicity: apart from a factor sensitive to the star’s composition (Ye being the ratio of the number of electrons to the number of nucleons), it contains only a numerical factor calculated from universal physical constants. For white dwarfs made up of C, O or Si, Ye = 0.5 and the mass limit is MCh = 1.4 M , but it is smaller for an iron-rich white dwarf, because then Ye = 0.464 and MCh = 1.2 M . (An iron white dwarf would form when a mainsequence star was massive enough, say 6–8 M , to create iron nuclei in its core but was able to lose mass and angular momentum e ectively to collapse into a white dwarf.) Sirius B and Eri B belong to the former group, whereas Procyon B and, perhaps, Stein 2051 appear to belong to the latter, as shown in Fig. 8.12.
|
40 Eri B |
|
|
|
|
.012 |
|
|
|
) |
|
|
|
|
(R/R |
Stein 2051 |
|
|
|
|
|
|
|
|
Radius |
|
Procyon B |
|
Sirius B |
.008 |
|
|
||
|
|
|
||
|
|
|
|
|
|
|
|
|
C |
|
|
|
|
Fe |
|
.004 |
|
|
|
|
0.4 |
0.6 |
0.8 |
1 |
|
|
|
Mass (M/M ) |
|
Figure 8.12: Mass–radius relation for white dwarfs and data for Sirius B, Eri B, Procyon B and Stein 2051 (based on J. Provencal, H. Shipman, E. Høg and P. Thejll, 1998).

8.4. White Dwarfs |
299 |
8.4.4 Luminosity
With a surface gravity 100 000 times that of the earth, the atmosphere of a white dwarf should have unusual properties. It consists of a very thin envelope, containing ordinary hydrogen and helium at the top and heavier elements at the bottom, wrapped around a 50 km thick rock-like crust. Underneath lies a central solid core made of carbon and oxygen atoms packed in extremely high densities.
The white dwarfs we know of have higher e ective temperatures than the sun, but, with much smaller radii, they are 1 000 times less luminous. As their outer layers remain non-degenerate, they radiate energy into space by photon di usion. But the situation changes completely in the interior. In general, absorption of light by matter takes place when the absorbing electrons can make a transition to a higher-energy state. But if matter is degenerate, as is the case of the white dwarf’s core, the Pauli exclusion principle makes such transitions impossible for all but those few electrons near the Fermi level, which alone are free to absorb photons and jump to higher empty levels. And so, most electrons whiz around for a long time before making any collisions and losing energy.
With the electron thermal energy essentially locked up, the only significant source of heat to be radiated is the ion thermal energy left over from the original gravitational collapse. This energy store is quite substantial (like 1040 J for T = 107 K), comparable to the radiant output of a supernova. White dwarfs emit radiation at the expense of their heat reserves, glow ever more faintly, cool down and, finally, come into thermodynamic equilibrium with the cold universe. In the process, as the internal temperature falls, the electric forces between the nuclei will eventually dominate the thermal motions, and the nuclei will begin to ‘sti en up’ under their mutual Coulomb repulsion. Thus, the white dwarf’s core crystallizes, consuming more heat from the star’s dwindling energy store.
As white dwarfs keep radiating weakly at decreasing rates through small constant surface areas, they will take billions of years to cool o and finally become black dwarfs, cold compact objects forever supported by the electron degeneracy pressure.16
8.4.5Summary
When a typical low-mass star reaches the end of its normal nuclear-powered evolution, it has lost most of its outer layers of material and becomes reduced to a
16Here, we are assuming isolated white dwarfs. When the white dwarf belongs to a binary or multiple system, it can accrete matter from a main-sequence companion that is going through the giant stage, and undergo recurrent outbursts (novae) which blow away its surface layers. If accretion is massive enough or lasts for a long time, the white dwarf may accumulate so much matter that it goes over the Chandrasekhar limit. The electron degeneracy pressure then gives way, and the star blows itself apart entirely in a thermonuclear blast, leaving no remnant core behind. This kind of event is called a Type I supernova, to distinguish it from Type II supernovae, the end state of a massive star described in the preceding section.

300 |
Bright Stars and Black Holes |
compact core containing still-hot but inert carbon. The core is supported against self-gravity by the degenerate-electron pressure in its interior, so that its mass and radius are related. However, for the degeneracy pressure to be e ective, its mass must not exceed 1.4 M . Although its residual internal energy is still substantial, an isolated white dwarf continuously radiates away this energy and becomes ‘black’ after billions of years.
8.5Neutron Stars
Neutron stars are the extremely dense compact objects left over from initially massive stars at the end of their normal evolution amid the violence of supernova explosions. Predicted by Walter Baade and Fritz Zwicky in 1934 and first studied by J. R. Oppenheimer and G. M. Volko in 1939, their existence was confirmed only in 1967, when Jocelyn Bell and Anthony Hewish detected a radio pulsar. Many others have been discovered since then; some are solitary, others have companions. Because of their extreme and complex physical properties, neutron stars manifest themselves in unusual and often unexpected guises, of which we only begin to have a cohesive picture, thanks largely to intensive computer simulations and abundant data sent back by a new fleet of orbiting astronomical satellites.
8.5.1 Formation and Structure
Once a star with an initial mass between 6 and 20 M has gone through the successive stages of nuclear burning and reached the final fusion phase, it keeps on accumulating iron in its center (see Section 8.3). Matter builds up so quickly that within a day or two, the iron central core goes over the Chandrasekhar mass limit, and the electron degeneracy pressure, which has so far successfully opposed the increasingly strong gravitational pull, suddenly fails, and the core begins to contract sharply.
As the internal pressure increases, it becomes energetically more favorable for the free degenerate electrons to be captured by nuclei, according to the basic equation e− + p → n + ν. The neutrinos thus produced scatter a bit, but quickly escape the interior and help the supernova happen. The reacting nuclei which stay behind get one of their protons replaced by a neutron and, after a few more captures, become neutron-rich, having more neutrons than they need for their stability. The newly created neutrons must go to higher and higher unfilled nucleon energy levels (because, having spin one-half, they must obey the Pauli exclusion principle), and so are less tightly bound than the neutrons already there. As the capture adds more and more neutrons to the nucleus, they will come closer and closer to the continuum (the region of unbound states), and some eventually begin to drip out (float out) of the nucleus as ‘free’ neutrons. Above a density of ρ ≈ 4 × 1014 kg m−3, the core

8.5. Neutron Stars |
301 |
consists of degenerate electrons and partially degenerate nucleons whizzing around the sluggish nuclei.
As the density reaches 5 × 1016 kg m−3, the neutrons have an average kinetic energy of about 8 MeV (the average nuclear binding energy) and can no longer be recaptured by nuclei. Matter is then composed of increasingly abundant free neutrons in addition to electrons and neutron-rich nuclei. The neutrons, which now are supplying a larger and larger part of the density and pressure, have become fully degenerate: their Fermi energy amply exceeds the thermal energy, even at the high temperatures (T ≈ 109 K) now prevailing in the star. When matter in the core becomes as dense as in a typical nucleus — that is, ρ ≈ 2.3 × 1017 kg m−3
— the nuclei begin to merge together, creating a unique state of nuclear matter composed chiefly of neutrons, with only a few percent of charged particles (protons and electrons). Each cm-cube of matter now contains 1041 neutrons, and the neutron degeneracy pressure becomes powerful enough to stop the collapse and support the core against its own weight. A violent shock-wave created by the sudden halt of the implosion travels outward, carrying with it the kinetic energy of the infalling material. It blows away the outer layers of the star, leaving behind a bared core packing a typical mass of 1.4 M into a sphere of only 10 km in radius: a neutron star is born.
Fascinating though a neutron star might be, you are well advised not to venture there. On the surface of the star, you would be crushed by a powerful gravitational force (100 billion times stronger than it is on earth) and vaporized by the intense heat (up to 106 K). The reduced size of the star also means that it rotates very fast (like 100 rotations per second) and is strongly magnetized (up to 108 tesla (1 T = 104 G), compared to 10−4 T for the sun). Most stars rotate and are magnetized. When such a star collapses spherically, it spins up (by a factor R−1 that increases with decreasing radius) to conserve angular momentum, and its magnetic field strength grows as R−2 to conserve the magnetic flux (which is ‘frozen’ by the ionized plasma).17 The intense magnetic lines which thread the resulting neutron star can influence its internal structure and the medium surrounding it.
So, what is a neutron star’s structure like? Imagine we start at the surface and burrow our way down, we will find the following zones (see Fig. 8.13):
(1)A thin surface layer, at densities of 109 kg m−3, which consists of atomic polymers of 56Fe plus a small number lighter elements in the form of a close packed solid. (Neutron stars are the only stars with a solid surface.) Atoms immersed in strong magnetic fields change drastically their energy states and take up cylindrical shapes aligned parallel to the field lines.
(2)An outer crust (1010 ρ 1014 kg m−3), which is a solid region composed of separate heavy nuclei forming a Coulomb lattice embedded in a relativistic degenerate electron gas, similar to the material found in white dwarfs. As the electrons can
17See Chapter 3.
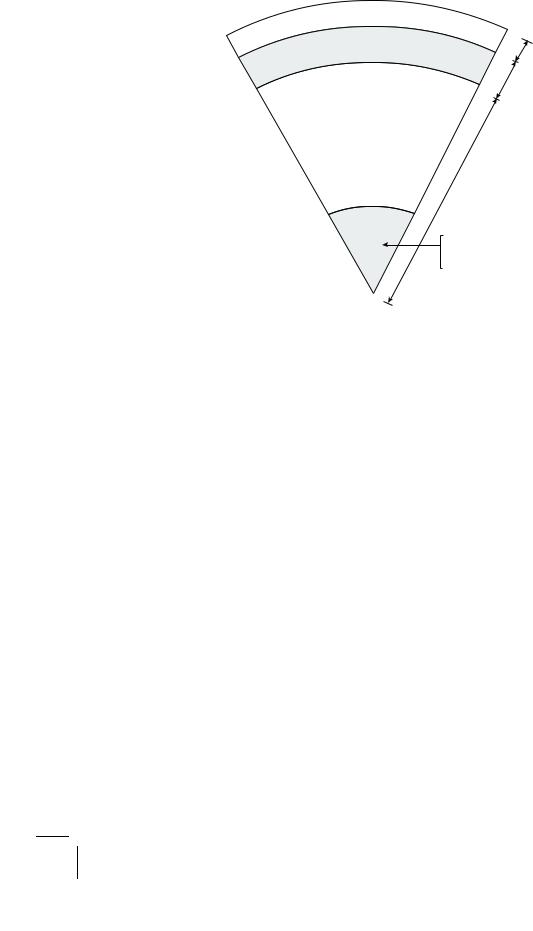
302 |
Bright Stars and Black Holes |
Nuclei, electrons
Nuclei, electrons, neutrons
0.3 km
0.5 km
Superfluid neutrons Super fluid protons electrons
9.5 km
? |
π Condensate |
|
quark |
Figure 8.13: Internal structure of a typical neutron star.
travel great distances before interacting, the electrical and thermal conductivities in this zone are enormous.
(3)When the density reaches 4 × 1014 kg m−3, it becomes energetically more favorable for neutrons to drip out of the nuclei and move around as free particles. As the density increases further, more and more of the nuclei dissolve, and the degenerate neutron gas provides most of the pressure.
(4)Deeper down, in a region that occupies 91% of the star’s volume, the neutron liquid phase occurs at densities above the nuclear density, and matter consists mainly of neutrons, with a 5–10% sprinkling of protons and electrons. The longrange attractive nuclear forces between neutrons grow su ciently strong to promote the formation of coherent pairs, and the neutron gas becomes superfluid. The protons undergo a similar phase transition to a superfluid and superconducting state. However, we expect the degenerate electrons to remain in the normal phase, their long-range coupling too ine ective to create favorable conditions for pairing.
(5)Finally, in the very center of the star, where the density may exceed 1018 kg m−3, all sorts of subnuclear particles will appear as products of reactions
between highly energetic electrons, protons and neutrons. Here, we may have really exotic matter, like hyperons and isobars, pion and kaon condensates. Or the mesons and hyperons may dissolve into quarks and form quark–gluon plasmas.
At least, that’s how theorists see it. The way to test their model is to examine their predictions on observable properties of neutron stars.

8.5. Neutron Stars |
303 |
First, the mass and radius, two fundamental parameters of any stellar objects. If we adapt the treatment for the degenerate electrons in Sec. 8.4 to the case of degenerate neutrons we have here, we will find that the maximum possible mass for a degenerate neutron star is 5 M . However, this estimate does not take into account general relativity, which would tend to lower the maximum mass because generalrelativistic gravitational forces inside compact stars are stronger than Newtonian gravitational forces, and so can overwhelm the quantum-mechanical degeneracy pressure at lower masses. General-relativistic calculations have led to the conclusion that the mass of a neutron star cannot exceed 3.2 M , independent of assumptions on the details of internal structure.
The actual value of the maximum mass is sensitive to the star’s assumed internal structure; calculations have it ranging between 1.5 and 2.7 solar masses, with the lower value corresponding to a core that contains hyperons and strange mesons. Now, whereas we cannot measure the radii of neutron stars with great certainty (although we expect them to be in the 10–13.5 km range), the masses of neutron stars (at least in binary systems) are the best known masses in astronomy: numerous recent data show that they all cluster around 1.4 M , with none above 2 M . This supports a lower value for the maximum possible mass (although its exact value is uncertain) and provides some evidence for the existence of a complex internal structure for the neutron stars.
Another way to verify the model is to examine the neutron star’s thermal history: how does it cool o ?
At the moment of the neutron star’s birth, its temperature may reach 1012 K, but it falls below 1011 K within seconds, and keeps falling fast. In this early epoch
(say, within the first 10 years), neutrinos are produced in abundance in the reactions n → p + e− + ν¯e, n + n → n + p + e− + ν¯e and n + p + e− → n + n + νe. They escape with little hindrance into space, carrying with them a huge amount of energy and thereby helping the star to cool down. When the temperature has dropped far enough (between 10 and 10 000 years after birth), processes less sensitive to temperature (such as thermal photon emission, thermal pair bremsstrahlung) take over, and the cooling process slows down. In this ‘standard cooling’ picture, a thousand-year old neutron star would have a surface temperature of a few 106 K.
But if the center of the star contains exotic stu — like strange matter, meson condensates or quark–gluon plasmas — then the neutrino cooling processes, which go with temperature as T 6, can operate for longer than we thought, which would cool things down very fast. In other words, as long as the center is made of exotic subnuclear particles, the interior heat drains o much faster than predicted by the standard cooling model.
The ‘observed’ thermal evolution of the neutron stars is obtained from the general assumption that all neutron stars are basically the same, and by making the correspondence of the star ages and core temperatures (the latter by extrapolating the surface temperatures, assuming isothermal interiors). Unfortunately, we have few cases where such data are available, and even in these, the experimental errors

304 |
Bright Stars and Black Holes |
are large. Nevertheless, the available data show deviations from the standard cooling, and provide some evidence of rapid cooling and, hence, the possible presence of an exotic core.
8.5.2Solitary Neutron Stars
How can you see a very dim object, twenty kilometers across and thousands of light years away? Nobody knew the answer until 1967, when it came in an unexpected way. Anthony Hewish and his team of astronomers in Cambridge, England, had just set up a radio telescope and were planning to observe the scintillations, or the rapid, irregular variations in the flux of radio waves from distant sources. The twinkling arises from irregular di raction of radio waves when the radiation passes through regions of inhomogeneous density in the plasma of the solar corona before reaching the earth. It can be observed only if the angular sizes of the sources are small enough, less than half a second. Hewish therefore decided to use the scintillation technique to study quasars, those extremely distant extragalactic radio sources whose angular sizes are measured in thousandths of a second. Up until then no detection devices had been built to measure short-time changes of radiation for wavelengths in the three-meter region of the radio spectrum. A young graduate student on his team, Jocelyn Bell, noticed something rather odd on the charts produced daily by the antenna. She found an unfamiliar point source that was scintillating even at night. More remarkably still, the flux did not fluctuate at random, as is the case with ordinary scintillations, but varied in a strictly periodic way. Very short pulses of radio waves, lasting about 50 milliseconds, were detected at very regular time intervals of approximately 1 second; the first pulsating emission source, or pulsar, was thus discovered.
Since then, more than 1 000 pulsars have been detected. Most exhibit broadband radio emission, a few are also seen in the X-ray or visible parts of the spectrum. The pulse intensities vary over a wide range, but the basic pulse is periodic. Pulsar periods are remarkably stable, except for a very slight overall increase over time. They lie in the 1.55 ms–5 s range; this means a pulsar with the shortest period is seen to flash strongly 660 times per second, whereas one with the longest period only once every five seconds.
But, how do we know that pulsars are neutron stars? As a bit of circumstantial evidence, some pulsars are in the middle of supernova remnants, where you would expect to find a neutron star. It is the case of the young pulsars in the Crab and Vela supernova remnants. (But you could not detect old pulsars in this way, because the remains of supernovas more than 105 years old would have entirely dissipated.) Further evidence comes from the brevity of the pulses. If a pulse of light lasts, say, 30 ms, it cannot come from an object more than 30 light-milliseconds (or 9 000 km) across. This limits us to white dwarfs, neutron stars, and black holes, which all could send periodic signals via vibrations, rotations or binary orbits. White dwarfs