
Invitation to a Contemporary Physics (2004)
.pdf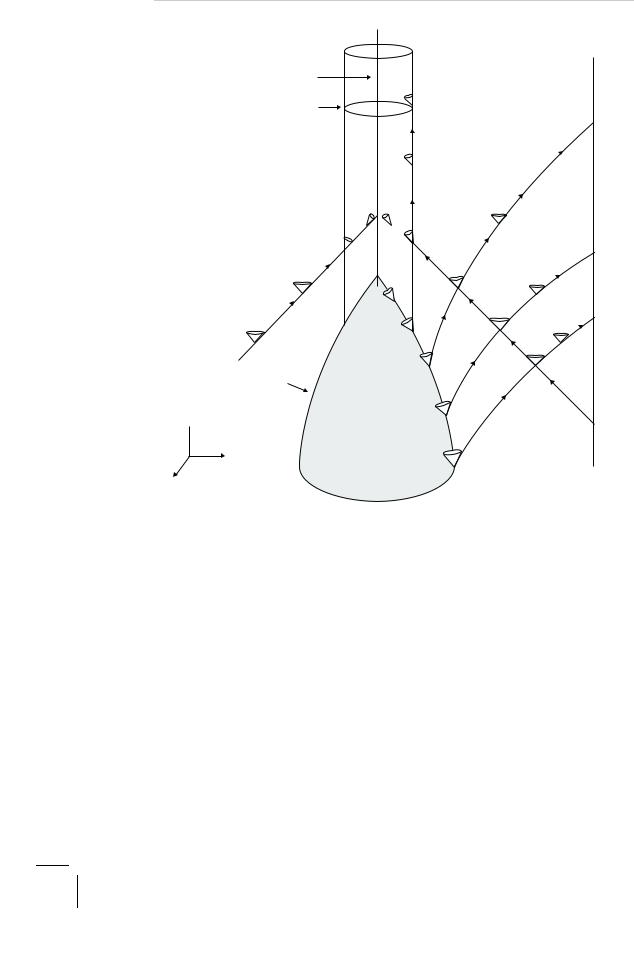
8.6. Black Holes |
315 |
r=0 |
Distant |
r=2M |
observer |
|
|
Singularity |
|
Event Horizon |
|
Outgoing light rays
Ingoing light rays
Collapsing star
Time
Space
Space
Figure 8.17: Gravitational collapse of a spherical star into a Schwarzschild black hole. Light rays in various directions of propagation are shown as successions of light cones.
get away are restricted to a cone aimed radially outward whose opening becomes narrower and narrower until only a pencil of vertical rays emerge free. On the horizon itself, it is possible for light to remain stationary, sandwiched between those signals just outside of the horizon and those just inside, and hovering in place forever. This is why the surface r = rg of a Schwarzschild black hole is also called a static limit.
Imagine you send toward a black hole a space observatory, programmed to transmit signals at equally spaced intervals (by its clock) at some fixed frequency (by its standard). So long as the space probe is at large distances from the compact star, we receive these signals at nearly equal intervals. But as the spacecraft approaches its target, we observe that the signals are arriving at more and more
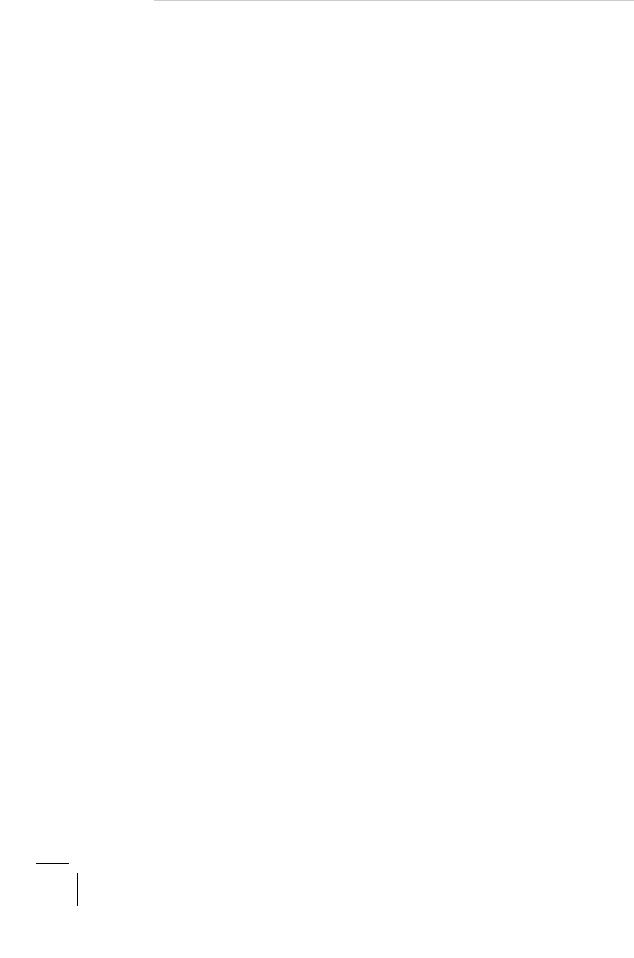
316 Bright Stars and Black Holes
widely spaced intervals and at wavelengths that become longer and longer. When the probe is close to the black-hole surface, the time dilatation and signal redshift thus observed increase exponentially with time by the earth-bound observer’s clock. The signal sent out just above the event horizon itself will take an almost infinite time to reach us, and will be infinitely redshifted when arrived. From our vantage, the space probe takes an infinitely long time to cross the Schwarzschild surface, and once it has crossed it, there is no way for us to learn directly what happens to it afterward. But for the spacecraft, nothing unusual happens as it passes the boundary, which it does smoothly and at a finite time by its clock. But once inside, it is pulled inexorably toward the central singularity, which it reaches in a few microseconds and where it disappears forever.
The most direct manifestation of gravitation and hence of spacetime curvature is the gravitational tidal e ect, which results from the non-uniformity of the gravitational field in spacetime. A familiar example is the tidal force of the moon, which causes the earth’s ocean tides. If an astrophysicist goes with the space mission we described earlier, tidal gravitational forces will stretch him from head to foot and, even worse, compress him on all sides. At some point, when the tension reaches 10 MPa (or 100 atm), his body will be broken. In a mission to a solar-mass black hole, this happens at 200 km from target, far outside the 3 km event horizon, but in an exploration of a supermassive 109 M black hole, the astrophysicist can survive up to a distance of 2 × 105 km from the center, far inside the critical radius of 3 × 109 km. As the spacecraft and its baryonic cargo cross the surface of no return and are propelled toward the center, they will be ripped apart and crushed by rapidly rising tidal forces. By the time the point of infinite curvature is reached, the mash of quarks to which the space mission has been reduced will be squeezed to zero volume and infinite density.
Let us turn now to real stars with asymmetries, magnetic moments, and possibly electric charges. The non-spherical collapse is a more complex process which we now understand well from results of a number of calculated examples and precise theorems. The key elements of spherical collapse — instability, implosion, the formation of an event horizon, the rapid decay to a ‘black’ stationary state, and the presence of some kind of singularity at the center — are believed to occur also in general non-spherical collapse. The main additional phenomenon is the emission of gravitational and electromagnetic radiation during the collapse.
The first local sign that a black hole is forming is the appearance of a trapped surface within the collapsing star; by this term, we mean a closed spatial surface (spherical or not) such that light rays emitted perpendicularly from the surface encounter enough matter or gravitation to converge, regardless of whether the rays are emitted in the outward or the inward direction. These converging rays will never reach infinity, and so the trapped surface lies inside an event horizon. For instance, any spherical surface inside the event horizon in the Schwarzschild geometry is a trapped surface; we know then that the convergence of light rays can be attributed to gravity, which pulls the photons to the central singularity. Stephen Hawking and

8.6. Black Holes |
317 |
Roger Penrose proved in the 1970s that a symmetric or an asymmetric spacetime that contains a trapped surface (and satisfies some general properties) must have a singularity, a region of spacetime where physical theory breaks down. In black holes, we interpret this singularity as a region of infinitely strong tidal forces. If one assumes (the Cosmic Censorship Hypothesis) that singularities resulting from gravitational collapse are hidden from view and hence causally cut o from the external universe, as is true in the spherically symmetric situation, then an event horizon will arise.
Once formed and left undisturbed, a black hole will settle down rapidly into a final stationary state characterized uniquely by the values of the mass M, spin J and charge Q of the hole (and no other attributes whatsoever: ‘a black hole has no hair’). This is the conclusion that suggests itself from a set of powerful theorems due to Hawking, Werner Israel and Brandon Carter. Why should M, J and Q determine completely the final state of the black hole? Because of all quantities intrinsic to an isolated source of gravity and electromagnetism, only the mass, spin and charge are locked to the distant external fields of the source (mass and spin to the gravitational field, charge to the electromagnetic field) by their relations to the conserved flux integrals over surfaces surrounding the hole and far from it. While the collapsing star quickly settles down to the unique configuration corresponding to the given M, J and Q, the fields undergo dynamic changes: all the higher-multipole asphericity in the gravitational field not compatible with M and J, and all electromagnetic moments, except the electric charge, detach themselves and blow o to infinity as radiation. The outcome of this process is a black hole with the specified final values of M, J and Q, and the external fields determined uniquely by those values. All other properties of matter, such as composition, baryon number and lepton number, are swallowed by the black hole, cut o from outside observations.
Black holes are unlikely to have a substantial charge. If a hole has any finite charge, the electric forces it exerts on a distant plasma would overwhelm gravitational forces, causing charge separation and pulling enough particles of opposite charge to the hole to neutralize it. By contrast, most stars that collapse rotate so fast that the black holes they produce have an angular momentum equal or nearly equal to the maximum allowed, Jmax = M2. Therefore, to all intents and purposes, all black holes in the universe have just two characteristics, mass and spin. Such a black hole is described by an exact solution to Einstein’s field equations, called the Kerr geometry (for Roy Kerr who created it). Listen to Chandrasekhar as he comments on this remarkable result: “(Black holes) are, thus, almost by definition, the most perfect macroscopic objects there are in the universe. And since the general theory of relativity provides a single unique two-parameter family of solutions for their description, they are the simplest objects as well.”
The Kerr black hole’s event horizon (the closed surface that lets no photons escape from inside) has a radius that depends on the angular momentum:
rg = [M + (M2 − a2)1/2] ,
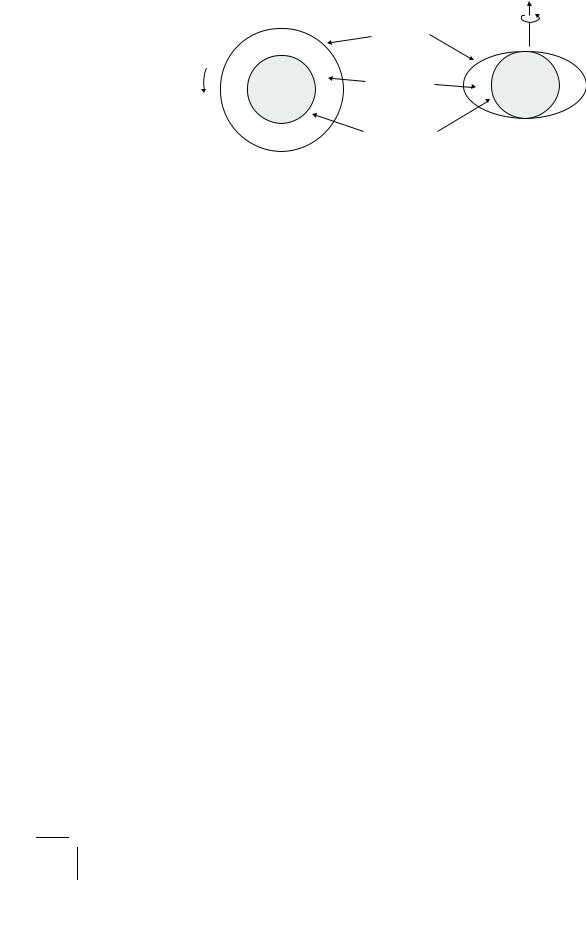
318 |
Bright Stars and Black Holes |
Static limit
Ergosphere
Event horizon
Top view |
Side view |
Figure 8.18: A Kerr black hole has a static limit and an event horizon; the region between these two surfaces is an ergosphere.
where a = J/M. It is smaller than the corresponding value for the Schwarzschild horizon and reduces to it when a = 0. (We are assuming that |a| ≤ M; should this not be the case, there will be no horizon and we shall have a naked singularity, in violation of the Cosmic Censorship Hypothesis.) The Kerr black hole is not spherically symmetric, only axially symmetric, because its static limit
r0 = [M + (M2 − a2 cos2 θ)1/2] ,
depends on a certain angle θ defined such that the ‘poles’ correspond to θ = 0, π, and the ‘equator’ to θ = π/2. This surface touches the horizon at the poles and intersects the equatorial plane on a circle whose radius is larger than that of the horizon (Fig. 8.18). On this surface, no particles can stay in the same place; only a light signal emitted in the radial direction can be at rest, and so must appear as infinitely redshifted to a far-away observer. For radii smaller than r0, but larger than rg, all matter, regardless of the forces acting on it, is dragged irresistibly around by the rotation of the black hole, although it can still, if powered properly, escape again to infinity. Only outside the static limit is it possible to surmount this dragging with a finite acceleration and remain fixed with respect to the distant universe.
8.6.2 Black Hole Dynamics
The space between the horizon and the static limit of a rotating black hole is called the ergosphere. One can in principle extract energy from the hole by having particles move in and out of this region. The key to this mechanism is that there are orbits in the ergosphere that support particles of negative total energy, meaning their gravitational binding energy exceeds the sum of their mass and kinetic energies. Imagine you send in from infinity a particle that splits into two parts in the ergosphere in such a way that one part, as judged by a far-away observer, follows a negativeenergy trajectory into the horizon, while the other part escapes to infinity with an

8.6. Black Holes |
319 |
energy that is in excess of that of the original particle, by conservation of the total energy. An energy has thus been e ectively extracted from the rotational energy. This process causes the black hole to spin down slightly and reduces its mass by an amount equivalent to the extracted energy. There is a limit to the amount of energy that can be extracted; it is given by ∆M = M − Mirr, where Mirr is the irreducible mass of the hole, defined such that
M2 = Mirr2 + J2/4Mirr2 . |
(8.8) |
When all the rotational energy has been extracted, we are left with a spherical black hole of mass Mirr, whose value cannot be further reduced by any interaction whatsoever. By the same token, a black hole can accrete matter selectively, in the direction of its own rotation, so that it can spin up to the maximum allowed spin, Jmax = M2 = 2Mirr2 , at which the rotational energy attains also its upper limit, consisting of 29% of the total energy.
One of the few known general properties of non-stationary black holes is the area theorem, which states that in any process involving black holes, the total surface area A of the horizons can never decrease; it can at best remain unchanged, when the conditions are stationary. We write the corresponding equation as dA ≥ 0, which is equivalent to dMirr ≥ 0 for arbitrary small changes in M and J. Equation (8.8) tells us that any decrease in M must come from a decrease in J and, furthermore,
Mhas Mirr as the lower limit.
The area theorem leads to a remarkable unification of thermodynamics and
gravity, which we will now discuss. The condition dA ≥ 0 points to a formal analogy between black hole area and entropy of a closed system: both tend to increase with time. The entropy of a black hole, SBH, must be a function of A with dimensions of Boltzmann’s constant k. So we write SBH = kf(A). The simplest choice is a linear function, that is, f(A) = bA/L2P, where b is a pure number, and LP any universal
length introduced to make f(A) dimensionless. If we take LP as Planck’s length
√
LP = = 1.61 × 10−35 m, then a calculation shows that b = 1/4. So, we have a very simple formula, SBH = kA/4 , for the entropy of any black hole of boundary area A. This result was first obtained by Jacob Bekenstein and Hawking. (It was re-derived in 1996 using string theory.)
What does this area–entropy relation mean? In statistical physics as in many other fields, entropy represents missing information. In black holes, it must represent all the information (hair) lost after the collapsing stars have settled down into stationary objects known to us only by the parameters M, J and Q. It represents the external observer’s deep ignorance about the star interior, about all the possible microstates of di erent temperature, shape, color, composition, etc. that contribute to making up a macrostate specified only by M, J and Q. The entropy quantifies this deep ignorance of ours about the physics under the horizon: exp(SBH/k) gives precisely the number of those possible microstates. By doing a quick calculation for a solar-mass black hole, you will see that SBH/k is a huge number, larger than the sun’s thermal entropy by a factor of 1020.
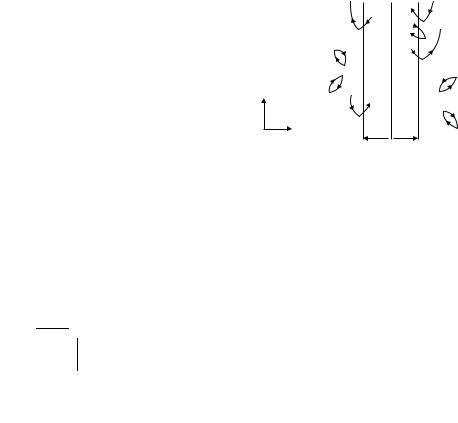
320 |
Bright Stars and Black Holes |
Briefly, the area theorem looks very much like a version of the second law of thermodynamics. We can also obtain an analog of the first law of thermodynamics, which gives the energy change of a closed system as it evolves from one state to a nearby state. It is, in fact, an energy conservation condition, which says that the energy change in the transformation, dE, is heat plus any work done on the system: dE = T dS + W . To simplify, we restrict ourselves to Schwarzschild black holes, in which case W = 0, A = 16π M2, and M = Mirr. (Generalization to include charge and rotation is straightforward.) Since we already know E = M and SBH = kA/4 , we easily obtain T = TH = /(8πkM). If we follow the thermodynamic analogy further, we must consider TH the black hole temperature. Note that, just as SBH is proportional to the black hole surface area A, so TH is proportional to its surface gravity, g = 1/(4M) (which is the acceleration, as measured at spatial infinity, of a free-falling test particle near the horizon); the proportionality factors in both cases contain only fundamental physical constants.
That a black hole behaves as a thermodynamic black body with entropy kA/4 and temperature g/(2πk) is rather puzzling, since we know that any object with a finite temperature must emit radiation, and black holes are absorbers rather than emitters. The missing piece of the puzzle was found by Hawking in 1974 when he discovered the thermal radiance of black holes.
The presence of the Planck constant in black-hole entropy and temperature indicates that these concepts must have quantum origins. According to quantum theory, a vacuum is not an absolute void, but a sort of reservoir of pairs of virtual particles and antiparticles of every kind (also known as ‘vacuum fluctuations’). These particles and antiparticles are considered ‘virtual’ because they can only appear transiently, for time intervals too short to measure. Such a pair, with energy ∆E, may be created out of the vacuum, in violation of the conservation of energy, provided it exists only for a short enough lapse of time, ∆t < /∆E, in accordance with the energy–time uncertainty relation.
Time
Space
Escaping antiparticle
Eventhorizon |
horizon |
|
Event |
Escaping particle
Figure 8.19: Hawking’s radiation. In this space-time representation, the black hole is propagating vertically in time at a fixed point in space. In the course of pair creation processes near the event horizon, some pairs are split up, one member falling into the black hole, the other escaping to infinity.

8.6. Black Holes |
321 |
Vacuum fluctuations may take place in any spacetime, including the vicinity of a black hole (Fig. 8.19). Consider, for instance, a fluctuation that produces two photons, one of energy E, the other with energy −E. In flat spacetime the negative-energy photon would not be able to propagate freely for long; it would recombine with the positive-energy photon within the time /E. But if produced just outside the horizon, it may cross the horizon before the time /E elapses; once inside the horizon, it can propagate freely along one of the negative-energy trajectories that exist inside the hole due to the large gravitational binding e ects, to vanish eventually at the singularity. Meanwhile, the positive-energy photon has a chance to escape to infinity as a free, real particle, carrying with it an energy E. According to quantum mechanics, we may view an antiparticle falling into the hole as a particle coming out of it but traveling backward in time. At the point where the pair was originally created, the particle is deflected by the gravitational field and moves forward in time. The particle that is escaping appears to a distant observer to have emerged from inside the black hole by tunneling through the gravitational barrier on quantum-mechanical principles.
Hawking found that the particles emitted in these processes have a thermal distribution of energies, with characteristic temperature given by the same formula as obtained above, TH = g/2πk (which holds even in the presence of charge and rotation, with appropriate g). This form of TH can be understood qualitatively by setting a thermal wavelength /kTH equal to the gravitational radius 2M. The numbers work out that TH ≈ 0.6 × 10−7(M /M) K: the bigger a black hole is, the cooler it is.
When a black hole emits particles, the outside world gains energy. By conservation of energy, there is a corresponding decrease in energy of the black hole, and hence a loss of its surface area, or entropy, in violation of the classical second law. But because of Hawking’s radiation, the entropy of the surrounding medium, Sext, must increase. Thus, the second law must be generalized to require that the total entropy, S = SBH + Sext, be a non-decreasing function of time.
We may readily estimate the power of this radiation: since rg = 2M and TH M−1, we have L rg2TH4 M−2. A solar-mass black hole would radiate at the rate of L ≈ 10−28 W in radio waves. The hole’s lifetime is thus limited by evaporation to τ M/L. Putting in all numerical factors, we have
τ = 1010 yr(M/1011 kg)3 .
This makes a solar-mass black hole live almost forever and its radiation unobservable. But black holes with much smaller masses could evaporate at a more perceptible rate. In particular, if small black holes (the primordial black holes) were formed at the beginning of the universe, those with masses greater than 1011 kg could have survived to this day; they would have the size of a proton and a temperature of 1011 K. At this temperature, they would emit a profusion of photons, neutrinos and gravitons; they would radiate at ever greater rates until they explode

322 |
Bright Stars and Black Holes |
violently out of existence. Mini black holes could last for some ten billion years, about the present age of the universe; so they could still be out there in space, sending out strong beams of very energetic gamma rays and waiting to be discovered.
8.6.3 Searching for Black Holes
Once formed, isolated stellar black holes do not emit light and would be hard to see. In principle, if a black hole is located on the line of sight of the earth to a distant star, its presence could be revealed by the gravitational lensing e ect, which refers to the large change in the apparent position of the star following the deflection of starlight by the strong gravity of an intervening compact object. But the probability for such an alignment is slim.
However, a black hole is never completely isolated; it is always surrounded by the interstellar medium, which it may capture. When matter falls down toward the horizon, it heats up by friction and emits thermal radiation. The radiation that escapes would be detectable at a distance. This radiation should be intense and more easily observable if there is a nearby rich source of gas or plasma, such as a companion star or a dense interstellar cloud. If the gas falls in radially, most energy goes down the hole in the form of internal energy or radial kinetic energy. But if it has a large spin, the gas will be captured first into a circular orbit, then gradually spiral down into smaller and smaller orbits while it sheds its excess of angular momentum. Matter circulating in these orbits forms a thin accretion disk around the black hole. The motion of particles at di erent speeds in adjacent bands of close orbits produces a viscous flow in the disk. It is this viscosity that transports angular momentum and energy outward, and heats the gas, ionizing it and causing thermal radiation. For a solar-mass black hole, temperatures as high as 108–109 K may be attained, and the disk radiates 10–100 keV X-rays.
The accreting process in black holes is not dissimilar to that occurring in neutron stars we described earlier, but two new features lead to distinctive observable e ects. The first is that the innermost edge of the accretion disk comes closer to the center, at the last stable orbit r rg. After passing this radius, matter spirals quickly inward with negligible further radiation. The total thermal energy liberated by matter as it traverses the width of the disk is just the gravitational binding energy in the innermost stable orbit. The second distinction is that the gravitational pull of very massive black holes may be so strong that the overflow of matter into the disk from the companion star might exceed the Eddington limiting value; only then will the maximum allowed amount manage to get down to the hole, while the rest will be forcefully expelled along the axis of rotation of the black hole in the form of two symmetrical powerful jets of hot gas, which can produce observable emission lines (Fig. 8.20). Needless-to-say, accretion disks around black holes are not well understood, being governed by the complexities of magnetohydrodynamics in general-relativistic settings. Calculations suggest that a thin disk of very hot
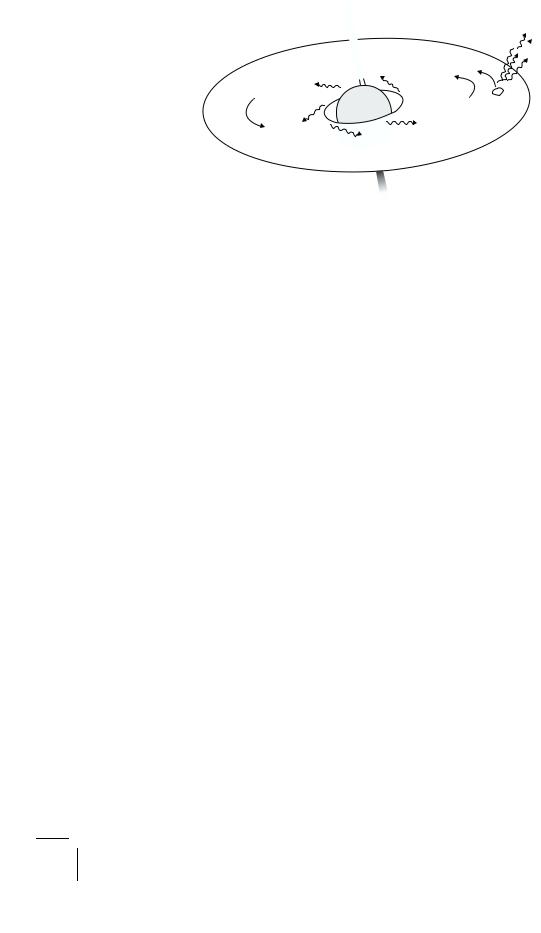
8.6. Black Holes |
323 |
Jet of hot gas
x-ray burst
Jet
Figure 8.20: Emission of high-energy particles and radiation by an accretion disk around a black hole.
gas is prone to local thermal instabilities. ‘Bubbles’ form from time to time at distances of several rg from the center, make several complete revolutions at a velocity approaching that of light, in millisecond periods, then dissolve. To a distant observer, they appear as highly variable bursts of nearly periodic fluctuations in the X-ray flux on millisecond time scales. The detection of these flux variations would provide strong evidence for the presence of a black hole and give the astronomer a good chance to measure the hole’s rotation.
So, how would you go about searching for stellar black holes? First, you would look for strong X-ray binary sources that are neither periodic nor recurrent. Then you would examine all the data available from spectroscopic observations of the companion star, assuming it to be optical; they may include the orbital period, amplitude of periodic Doppler shifts that characterize the spectroscopic lines of binary systems, reddening of light by interstellar dust, e ective temperature, and brightness. These parameters will allow you to determine a range of values for the mass of the compact star. If the lowest value turns out to be well above 3 M , a conservative estimate for the upper limit of the neutron-star mass, then you might have a black hole. And you would have a stronger case if you can show that the X-ray flux exhibits rapid variations, or the spectrum contains emission lines, which are the signature of ultra-relativistic jets.
The strongest existing case for a stellar black hole is Cygnus X-1, which has been intensively studied ever since its discovery in 1965. It is a powerful X-ray source that varies so rapidly that it has to be a compact object. Its companion is a hot blue giant with a surface temperature of 31 000 K and a mass in the 24–42 M range (most probably 33 M ). Spectral analyses yield an orbital period of 5.6 days and orbital size of 30 million km. From the star’s apparent magnitude, corrected by interstellar reddening, its distance should be 2.3 kpc. The absence of eclipses means that the inclination angle between the orbital plane and the direction of observation

324 |
Bright Stars and Black Holes |
exceeds 55◦. A careful analysis of these data sets a lower limit of 7 M and assigns a most probable mass of 16 M for Cyg X-1. Since this is well above the maximum allowed mass of neutron stars, Cyg X-1 must be a black hole.
We now know of many black-hole candidates in binary systems and several isolated black-hole candidates with masses in the 3–30 M range.24
As already mentioned, the theory of gravitational collapse sets no limits on the final masses, and one could imagine black holes of a thousand, a million or even several billion solar masses. In fact, it has been known for some time that supermassive black holes of 106–109 M exist in the centers of many galaxies, including our own. They could have developed by condensation of lumps in the early universe, or by gravitational collapse of a star-rich globular cluster, or gradual growth of a seed black hole in a very dense and confined environment. Black holes in the 400–105 M mass range have been found in galactic cores or star clusters; they could act as the seeds for future supermassive black holes.
Our understanding of black hole astrophysics has progressed at a rapid pace, matched by parallel technological advances, which allow construction of large X-ray telescopes, more sensitive gamma-ray detectors and very long baseline radio observatories capable of excellent angular resolution. These technologies will be harnessed in a network of ground-based observatories under construction and a new array of orbiting astronomical satellites, which can make detailed studies of black holes over a large energy range. They will give astronomers tools of unprecedented sophistication to study not only electromagnetic radiation, but also gravitational waves, which are the unambiguous signature of black holes.
8.6.4Summary
When massive stars become so compact and the gravitational force of gravity becomes so strong that no physical force can oppose total collapse, the final stationary state is a black hole. It is ‘black’ because light sent out from a limiting surface, called the static limit, will take an infinite time to reach a distant observer and will be infinitely redshifted when received. It is a ‘hole’ because light or matter falling within another surface, called the event horizon, cannot escape outside and, once inside, will be inexorably pulled to a central singularity, a point where the gravitational force is infinitely strong.
Astronomers have observed many compact objects in the 3–30 M mass range thought to be the final black-hole states of massive stars. But there are also compact, supermassive conglomerates in the 400–105 M , and even 106–109 M , mass range. These are thought to have developed by condensation of lumps in the early universe, or by gravitational collapse of star-rich globular clusters, or gradual growth of seed black holes in a very dense and confined environment.
24The website http://www.johnstonarchive.net/relativity/bhtable.html gives complete lists of black-hole candidates.