
Учебники / Genetic Hearing Loss Willems 2004
.pdf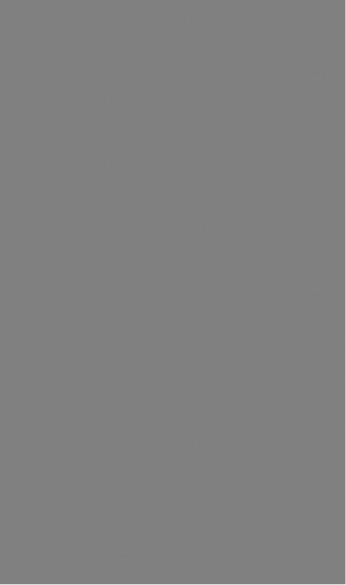
154 |
Dixon and Dixon |
Figure 1 A patient with Treacher Collins syndrome. (A) A lateral view of the patient illustrates the mandibular hypoplasia and the abnormal external ear. (B) A frontal view of the same individual illustrates the colobomas (arrows) and paucity of eyelid lashes on the medial side of the abnormality. Immediately beneath the eyes, some ‘‘flattening’’ of the zygomatic complex is visible.
Treacher Collins Syndrome |
155 |
sloping of the palpebral fissures (89%), frequently with colobomas of the lower eyelids and a paucity of lashes medial to the defect (69%), are also frequently seen (Fig. 1). Hypoplasia of the facial bones, particularly a ecting the mandible (78%) and zygomatic complex (81%), is also observed in TCS (Fig. 1). In severe cases, the zygomatic arch may be absent (10) and cleft palate (28%) may occur. These features are usually bilaterally symmetrical (Fig. 1) (11).
Although the genetic mutations underlying TCS are highly penetrant, the condition is characterized by marked interand intrafamilial phenotypic variability (7,12). Some individuals are so mildly a ected that it can be extremely di cult to establish an unequivocal diagnosis and to provide accurate genetic counseling on clinical grounds alone. Indeed, some mildly a ected TCS patients may only be diagnosed after the birth of a more severely a ected child. At the other end of the phenotypic spectrum, severe cases of TCS have occasionally resulted in perinatal death due to a compromised airway (13).
III.DIFFERENTIAL DIAGNOSIS
In the di erential diagnosis of TCS one should consider those conditions that form part of the oculoauriculovertebral (OAV) spectrum. This is a complex and heterogeneous set of conditions that includes hemifacial microsomia, a condition that primarily a ects development of the ears, mouth, and mandible. Like TCS, hemifacial microsomia shows marked clinical variability but, unlike TCS, it usually a ects one side of the face only. In cases where bilateral involvement has been described, there is generally marked asymmetry in the extent to which the di erent sides of the face are a ected (14). Goldenhar syndrome is also considered to be part of the OAV spectrum but includes vertebral anomalies and epibulbar dermoids in addition to the facial involvement. In most cases, OAV spectrum occurs sporadically, and the condition therefore tends to be characterized by a low (empirical) recurrence risk. However, as 1–2% of cases have a previous family history with autosomal dominant and autosomal recessive inheritance being implicated in di erent cases, counseling should be provided on an individual family basis. Other conditions in which the facial gestalt may resemble TCS include Nager acrofacial dysostosis and Miller syndrome, but in both cases there are accompanying limb abnormalities. While OAV, Nager, and Miller syndrome are usually readily distinguished from TCS, caution should be exercised where individuals are a ected minimally to ensure that these various conditions are not misdiagnosed.
156 |
Dixon and Dixon |
IV. IDENTIFICATION OF THE GENE MUTATED IN TCS
The combined facts that TCS exhibits a well-defined mode of inheritance and the majority of cases can be diagnosed clinically ensured that the gene mutated in this condition could be isolated using a positional cloning strategy, i.e., on the basis of its position in the human genome alone (reviewed in Ref. 15). Using a series of clinically well-characterized families, the gene mutated in TCS, TCOF1, was initially mapped to a 9 cM region of human chromosome 5q31–q34 using restriction length fragment polymorphisms (16). Subsequent studies concentrated on the use of highly informative short tandem repeat polymorphisms to refine the location of the TCOF1 critical region to a small genetic interval of chromosome 5q32–q33.1 (17–20). This region was then cloned using a combination of yeast artificial chromosome and cosmid clones (21–23), which were subsequently used in transcript mapping techniques to identify candidate genes that were screened for TCS-specific mutations. This strategy resulted in the identification of TCOF1 (24).
V.CHARACTERISATION OF TCOF1 AND TREACLE
TCOF1 encodes a low-complexity 1411-amino-acid protein, named Treacle, in which serine, alanine, lysine, proline, and glutamic acid account for over 60% of residues (25,26). Bioinformatics analyses have indicated that Treacle contains three domains: unique amino and carboxy termini, and a characteristic central repeat domain (25,26). The central repeat domain consists of 10 repeat units, each of which is encoded by an individual exon (exons 7–16). A single repeat consists of a cluster of acidic amino acid residues, containing numerous consensus sites for casein kinase II (CKII) phosphorylation, separated by basic amino acids comprising a majority of lysine, alanine, and proline residues. Further analysis of Treacle identified a number of potential monopartite nuclear localization signals (NLSs) of the consensus sequence K-K/R-X-R/K (27), embedded in the lysine-rich carboxy terminus of the protein.
Database searches revealed that Treacle does not exhibit strong homology to any previously identified genes or gene families. Such searches did, however, indicate that Treacle exhibits weak homology to rat nucleolar phosphoprotein Nopp140, with the greatest homology arising in the region of the repeated domain. These results have suggested that Treacle may also be a nucleolar protein (25,26). This hypothesis has been verified by subcellular localization studies using green fluorescent protein (GFP) reporters and anti-Treacle antibodies. These combined experiments demonstrated that the NLSs at the carboxy terminus of Treacle are functional and that Treacle localizes to the nucleolus (28,29). More recently, the subnucleolar local-
Treacher Collins Syndrome |
157 |
ization of Treacle has been refined and the expression domain found to lie within that of nucleolin, precisely mirroring that of fibrillarin, suggesting localization to the dense fibrillar component of the nucleolus (30). However, unlike Nopp140, Treacle is absent from the coiled (Cajal) bodies.
Treacle has been further characterized using biochemical techniques. Western blotting indicated that anti-Treacle antibodies recognized a single protein band of approximately 220 kDa in cell lysates (30). However, as the predicted molecular weight of Treacle is 144 kDa, in vitro transcription and translation studies were used to confirm that this is the correct molecular weight of Treacle as determined by sodium dodecyl sulfate–polyacrylamide gel electrophoresis (SDS-PAGE) analyses. Isaac and co-workers noted that the discrepancy between the theoretical molecular weight of Treacle and its mobility on SDS-PAGE was reminiscent of that of Nopp140 (30). As most of the aberrant mobility of Nopp140 is due to phosphorylation in its central repeat domain, which shares homology with that of Treacle, in vitro translated Treacle was treated with alkaline phosphatase in the presence or absence of phosphatase inhibitors. Treacle, like Nopp140, showed a f40-kDa mobility shift upon phosphatase treatment and migrated at f180 kDa, indicating a high degree of phosphorylation (30). Subsequently, it has also been determined that CKII and Treacle coimmunoprecipitate from HeLa cell lysates and that CKII is likely to be the kinase responsible for the posttranslational modification of Treacle (30). This is consistent with the results of Jones and colleagues, who showed that avian branchial arches contain a kinase activity that can phosphorylate Treacle peptides consistent with CKII site recognition (31).
Recently, another motif has been documented within the sequence of Treacle, a LIS1 homology (LisH) motif at amino acid positions 5–38 (32). The LIS1 gene is mutated in patients with Miller-Dieker lissencepahly (33). In this bioinformatics-based study, over 100 eukaryotic intracellular proteins were found to contain a LisH motif and from their diverse functions the authors suggested that these motifs might contribute to the regulation of microtubule dynamics, either by mediating dimerization or by binding cytoplasmic dynein heavy chain or microtubules directly. Currently, there have been no laboratory-based studies that support this hypothesis; however, it is interesting that Nopp140 also contains a LisH motif, reinforcing previous observations that Treacle and Nopp140 share structural and functional similarities.
VI. MUTATION ANALYSIS OF TCOF1
To date, mutation analysis of TCOF1 has resulted in the identification of over 120 mutations that are spread throughout the gene (24,26,34–36). While
158 |
Dixon and Dixon |
the mutations include splicing mutations, insertions, and nonsense mutations, by far the majority (68%) are deletions, which range in size from one to 40 nucleotides. In any event, the result of these mutations is to introduce a premature termination codon into Treacle, which suggests that the mechanism underlying TCS is haploinsu ciency. Although the majority of mutations tend to be family-specific, the recurrent mutation nt4135 del(GAAAA), which occurs in exon 24 of TCOF1, accounts for 18.6% of mutations.
A small number of putative missense mutations have also been identified in TCOF1 (34,36). Although one of these changes (K749K) initially appeared to be silent, it resulted from a G>A transition in the last nucleotide of exon 14. Unusually, the sequence of the associated splice donor site of intron 14 was found to be gc rather than the more usual gt, a variation that is present in the human, mouse, and dog TCOF1 genes (25,26,37,38). In vitro studies have shown that gc is the only substitution that will allow the 5V splice site to be accurately cleaved, although in such cases the surrounding sequence is usually very closely matched to the consensus AG/gcaagt (39). In the case of the mutation K749K, RT-PCR analysis indicated that the two variations from the splice consensus sequence resulted in abnormal splicing with readthrough into intron 14. As this mutation also results in the introduction of a termination codon, the net e ect is likely to be the same as the mutations outlined above (34).
The remaining missense mutations I14F, A41V, W53C, and W53R occur in the amino terminus of Treacle. Although their e ect on protein function remains unknown, it is noteworthy that this is the most highly conserved region of the protein (37,38,40). Moreover, although a high frequency of single nucleotide polymorphisms (SNPs) has been observed in TCOF1, no SNPs have been documented in that region of the gene encoding the amino terminus of Treacle in over 100 normal individuals (24,26,34,36). Nevertheless, until the exact function of Treacle is determined, and, in particular, the role that the unique amino and carboxy termini play in contributing to that function, these missense changes should only be considered ‘‘putative’’ mutations.
VII. MOLECULAR DIAGNOSIS OF TCS
Prior to the commencement of molecular studies on TCS, prenatal diagnosis was only possible using fetoscopy or ultrasound imaging and both methods have been used to make positive predictions (41–44). While the quality of ultrasound imaging has improved markedly in recent years, allowing noninvasive prenatal diagnosis to be performed, it may still be di cult to make a positive diagnosis, particularly where the fetus is mildly a ected. Given
Treacher Collins Syndrome |
159 |
these circumstances the procedure is usually not diagnostic for apparently una ected fetuses. Moreover, prenatal diagnosis using either fetoscopy or ultrasound imaging is not possible until the second trimester of pregnancy. Conversely, molecular diagnosis can be undertaken in the first trimester of pregnancy. Initially, this was achieved using linked polymorphic markers (13). More recently, in light of the identification of TCOF1, these predictions are now more reliable, since the presence or absence of a particular mutation can be assessed. However, genetic counseling of families with TCS is complicated by the fact that, in general, mutations between families are di erent (see above). Hence, the specific mutation must first be identified within a family before any counseling or prenatal diagnosis can be performed. Since this may require analysis of all 26 exons of TCOF1, it can be a time-con- suming process. In addition, even if the fetus is found to carry the diseasecausing mutation, no conclusions can be drawn about the severity with which the child will be a ected. Therefore, parents may opt to delay any decision making until ultrasound can be used to provide further information on the extent of the abnormalities. However, for those cases in which the fetus does not carry the mutation, parents can be reassured that their child will be una ected. The area in which molecular diagnosis is likely to find its greatest utility is in postnatal diagnosis of ‘‘at-risk’’ individuals. This will be particularly important in confirming the clinical diagnosis in mildly a ected individuals and also in accurately counseling apparently una ected parents of a child in which TCS has supposedly arisen as the result of a de novo mutation. Finally, it should be emphasized that the molecular diagnosis, although providing a useful adjunct to patient management in a subset of cases, is no substitute for careful patient examination leading to an accurate clinical diagnosis.
VIII. THE ROLE OF TCOF1/TREACLE IN
CRANIOFACIAL DEVELOPMENT
To investigate the developmental mechanisms underlying TCS, research has largely focused on the mouse. Although the human and murine proteins display relatively low homology, 54.8% identity, and 71.6% similarity, their orthologous nature has been confirmed by genetic mapping (37,40). Expression analyses have further shown that, although the gene is widely expressed, the highest expression levels are observed in the neural folds immediately prior to fusion, and in the developing first pharyngeal arch (37). These observations are consistent with a role for the gene during craniofacial morphogenesis. These studies laid the foundations for the use of genetargeting technology to study the function of the protein in vivo. To ensure
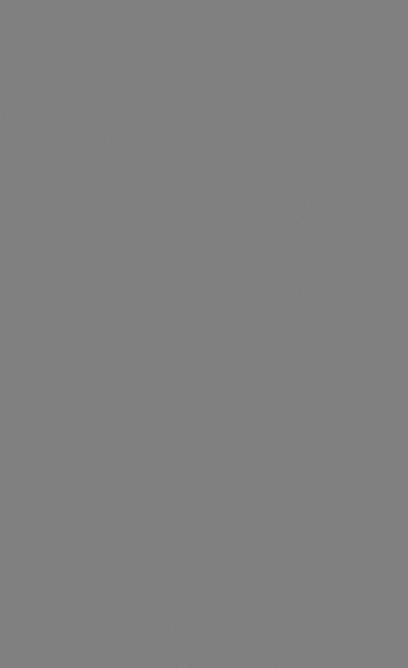
160 |
Dixon and Dixon |

Treacher Collins Syndrome |
161 |
complete functional inactivation of Tcof1, Dixon and co-workers replaced exon 1, which contains the translation initiation codon, with a neomycin resistance cassette via homologous recombination in embryonic stem cells (45). The chimeric animals generated using these cells were intercrossed with C57BL/6 female mice. Confirmed Tcof1 heterozygous (Tcof1+/ ) mice were found to die shortly after birth as a result of severe craniofacial anomalies that include agenesis of the nasal passages, abnormal development of the maxilla, acrania, exencephaly, and anophthalmia (45).
Developmental analysis showed that abnormalities were first detected in Tcof1 heterozygous mice at embryonic day 8 (E8), after which they exhibited a generalized developmental delay. At E8.5, wild-type embryos displayed welldeveloped head folds and the first signs of optic development, whereas Tcof1+/ embryos exhibited smaller, more rounded neural folds and an absence of the optic evagination. While all wild-type embryos had completed the axial turning sequence and closed their rostral neuropore by E9, their Tcof1+/ littermates remained unturned with a patent rostral neuropore. By E10.5 the formation of the olfactory pit in wild-type mice divided the frontonasal mass into medial and lateral nasal processes. In contrast, Tcof1+/ embryos failed to develop either of these processes, and exhibited exencephaly with neuroepithelium protruding through the rostral neuropore (Fig. 2A–D). By E14.5, the lack of nasal passages and anophthalmia in Tcof1+/ mice were clearly apparent. Mutant mice also displayed mandibular hypoplasia, severe retrognathia of the middle third of the face, and low-
Figure 2 (A and B) Scanning electron microscope analysis of E12.5 wild-type and Tcof1+/ embryos, illustrating severe abnormalities of the developing facial complex, including underdevelopment of the brain, anophthalmia, and abnormal nasal passages. (C and D) Histological appearance of E12.5 wild-type and Tcof1+/ embryos. Parasagittal sections reveal underdevelopment of the entire otocyst as well as the lack of an endolymphatic appendage in the Tcof1+/ embryo. The trigeminal ganglion is severely hypoplastic in the Tcof1 heterozygote. (E and F) Skeletal analysis of the skulls of postnatal wild-type and Tcof1+/ mice. The majority of the abnormalities are observed in the craniofacial region, including the lack of the frontal, parietal, and interparietal bones of the vault of the skull, as well as the abnormal nasal complex. (G and H) Whole-mount TUNEL analysis of wild-type and Tcof1+/ embryos at E8.5. A section through the neural folds of the wild-type embryo reveals infrequent apoptotic nuclei along the edges of the neural folds, whereas the Tcof1 heterozygous embryo exhibits massively elevated levels of apoptotic nuclei throughout the entire neuroepithelium, particularly in the lateral regions. e, ear; f, frontal bone; fb, forebrain; mb, midbrain; hb, hindbrain; fb/mb, forebrain/midbrain mass; m, mandible; mp, mandibular process; nc, nasal complex; nf, neural fold; oc, otocyst; p, parietal bone; tgg, trigeminal ganglion.
162 Dixon and Dixon
set cup-shaped ears. Skeletal analysis of E18 Tcof1+/ mice revealed an absence of the frontal, parietal and interparietal bones of the vault of the skull. The nasal capsule and the maxilla were grossly malformed. The zygomatic arch, the tympanic ring, and the middle ear ossicles were also hypoplastic and misshapen in Tcof1+/ mice (Fig. 2E,F) (45).
As the phenotype suggested an abnormality of neural crest cell development, whole-mount TUNEL analysis, a technique that identifies cells undergoing apoptosis, was used by Dixon and co-workers (45). This technique revealed that the number of apoptotic cells was markedly elevated in the Tcof1 heterozygous embryos; in particular, the neuroepithelium of the cranial neural folds and the neural tube displayed a profusion of apoptotic cells (Fig. 2G,H). These abnormally high levels of apoptosis were observed in Tcof1 heterozygous mice throughout embryonic days 8 and 9, but thereafter they declined. The high levels of cell death in the neuroepithelium, together with the gross morphological phenotype, strongly suggested that a proportion of the premigratory neural crest cells were depleted. In addition, immunohistochemistry of embryonic day10.5 mice with an antineurofilament antibody indicated that the neural crest cell-derived cranial ganglia were severely hypoplastic, the ophthalmic branch of the trigeminal nerve and the glossopharyngeal ganglia/nerves were absent, and the dorsal root ganglia were markedly disorganized. These results suggest that the TCS phenotype results, at least in part, from a massive increase in apoptosis in the prefusion neural folds.
Subsequent studies in our own laboratory have investigated the contribution of di erent genetic backgrounds to the phenotype of the heterozygous mice. To date, the Tcof1 chimeras have been crossed with females from di erent inbred strains. Although these studies are currently ongoing, it is clear that the genetic background on which the Tcof1 mutation is placed is a key determinant in the severity of the resulting phenotype. Continued investigation of the contribution of the genetic background to phenotypic variation in mice may, in the long term, help us to understand the basis of the marked interand intrafamilial variation observed in TCS families.
REFERENCES
1.Thompson A. Notice of several cases of malformation of the external ear, together with experiments on the state of hearing in such persons. Monthly J Med Sci 1846; 7:420.
2.Treacher Collins E. Cases with symmetrical congenital notches in the outer part of each lid and defective development of the malar bones. Trans Ophthalmol Soc UK 1900; 20:190–192.
Treacher Collins Syndrome |
163 |
3.Franceschetti A, Klein D. Mandibulo-facial dysostosis: new hereditary syndrome. Acta Ophthalmol 1949; 27:143–224.
4.Rovin S, Dachi SF, Borenstein DB, Cotter WB. Mandibulofacial dysostosis, a familial study of five generations. J Pediatr 1964; 65:215–221.
5.Fazen LE, Elmore J, Nadler HL. Mandibulo-facial dysostosis (Treacher Collins syndrome). Am J Dis Child 1967; 113:406–410.
6.Jones KL, Smith DW, Harvey MA, Hall BD, Quan L. Older paternal age and fresh gene mutation: data on additional disorders. J Pediatr 1975; 86:84–88.
7.Marres HAM, Cremers CWRJ, Dixon MJ, Huygen PLM, Joosten FBM. The Treacher Collins syndrome: a clinical, radiological and genetic linkage study on two pedigrees. Arch Otol 1995; 121:509–514.
8.Stovin JJ, Lyon JA, Clemens RL. Mandibulofacial dysostosis. Radiology 1960; 74:225–231.
9.Phelps PD, Poswillo D, Lloyd GAS. The ear deformities in mandibulofacial dysostosis (Treacher Collins syndrome). Clin Otolaryngol 1981; 6:15–28.
10.Poswillo D. The pathogenesis of Treacher Collins syndrome (mandibulofacial dysostosis). Br J Oral Surg 1975; 13:1–26.
11.Kay ED, Kay CN. Dysmorphogenesis of the mandible, zygoma, and middle ear ossicles in hemifacial microsomia and mandibulofacial dysostosis. Am J Med Genet 1989; 32:27–31.
12.Dixon MJ, Marres HAM, Edwards SJ, Dixon J, Cremers CWRJ. Treacher Collins syndrome: correlation between clinical and genetic linkage studies. Clin Dysmorph 1994; 3:96–103.
13.Edwards SJ, Fowlie A, Cust MP, Liu DTY, Young ID, Dixon MJ. Prenatal diagnosis in Treacher Collins syndrome using combined linkage analysis and ultrasound imaging. J Med Genet 1996; 33:603–606.
14.Gorlin RJ, Cohen MM, Levin LS. Syndromes of the Head and Neck. Oxford: Oxford University Press, 1990.
15.Dixon MJ. Treacher Collins syndrome. Hum Mol Genet 1996; 5:1391–1397.
16.Dixon MJ, Read AP, Donnai D, Colley A, Dixon J, Williamson R. The gene for Treacher Collins syndrome maps to the long arm of chromosome 5. Am J Hum Genet 1991; 49:17–22.
17.Jabs EW, Li X, Coss CA, Taylor EW, Meyers DA, Weber JL. Mapping the Treacher Collins syndrome locus to 5q31.3–q33.3. Genomics 1991; 11:193–198.
18.Dixon MJ, Dixon J, Raskova D, Le Beau MM, Williamson R, Klinger K, Landes GM. Genetic and physical mapping of the Treacher Collins syndrome locus: refinement of the localisation to chromosome 5q32–q33.2. Hum Mol Genet 1992; 1:249–253.
19.Dixon MJ, Dixon J, Houseal T, Bhatt M, Ward DC, Klinger K, Landes GM. Narrowing the position of the Treacher Collins syndrome locus to a small interval between three new microsatellite markers at 5q32–q33.1. Am J Hum Genet 1993; 52:907–914.
20.Loftus SK, Edwards SJ, Scherpbier-Heddema T, Buetow KH, Wasmuth JJ, Dixon M. A combined genetic and radiation hybrid map surrounding the Treacher Collins syndrome locus on chromosome 5q. Hum Mol Genet 1993; 2:1785–1792.