
Davis W.A.Radio frequency circuit design.2001
.pdf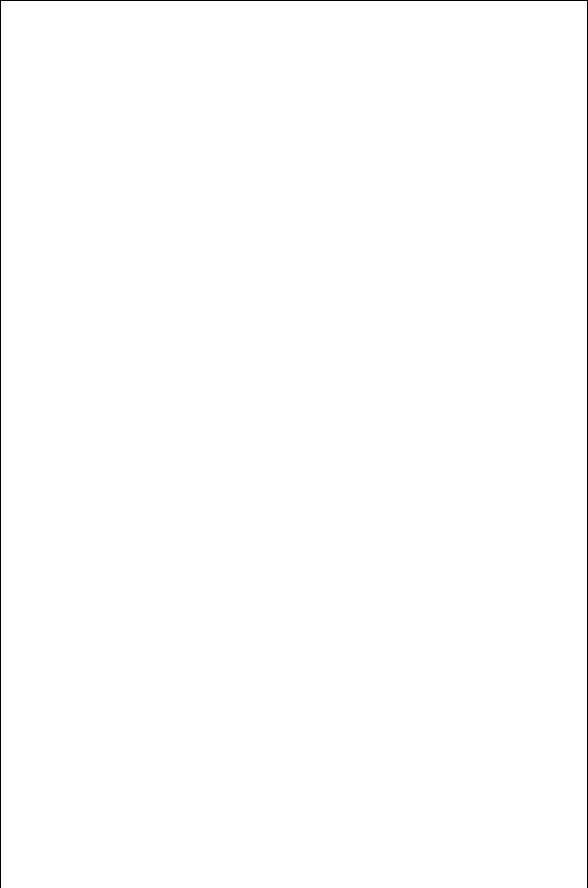
30 RESISTORS, CAPACITORS, AND INDUCTORS
and odd-mode capacitances are added together in proportion to their evenand odd-mode voltages:
CL D 0 C %Co Ð Cga C Cge |
2.59 |
Cqi D %Ce Ð Cf0 C Cm C Cf C %Co Ð Cm C Cf0 |
2.60 |
Detailed formulas for the circuit elements in Fig. 2.13 are found in [19] and some of these are summarized in Appendix B.
Once the equivalent / circuit for the spiral section is known, the entire spiral inductor is modeled by cascading each of these sections. The CL, R, and L are combined into the single impedance Zp:
Z |
|
R C sL |
2.61 |
|
p D s2LCL C sCLR C 1 |
||||
|
|
The ABCD parameters described in Chapter 4 (Section 4.2) are used to cascade the individual / circuits. Thus
A D 1 C sCq2Zp |
2.62 |
B D Zp |
2.63 |
C D s2Cq1Cq2Zp C s Cq1 C Cq2 |
2.64 |
D D 1 C sCq1Zp |
2.65 |
Each section of the spiral described in terms of an ABCD matrix may be cascaded together by simply multiplying ABCD matrices. The C in Eq. (2.64) is a matrix element, not a capacitance. Once the total cascaded ABCD matrix is found, the input impedance may be determined:
Z |
AZ L C B |
2.66 |
|
CZ L C D |
|||
in D |
|
The ZL is the load impedance on the output side of the spiral. If ZL is a short to ground, then the effective inductance of the spiral might be estimated by
L |
eff D |
=fZing |
2.67 |
|
ω |
||||
|
|
In the cascaded analysis the capacitance, Cq2, from one section is the same as the Cq1 of the subsequent section, and hence it ought not to be counted twice in evaluating the cascaded equivalent circuit. One approach is to simply choose Cq2 ! Cq2/2 and Cq1 ! Cq1/2, except of course for the innermost and outermost coupled line.
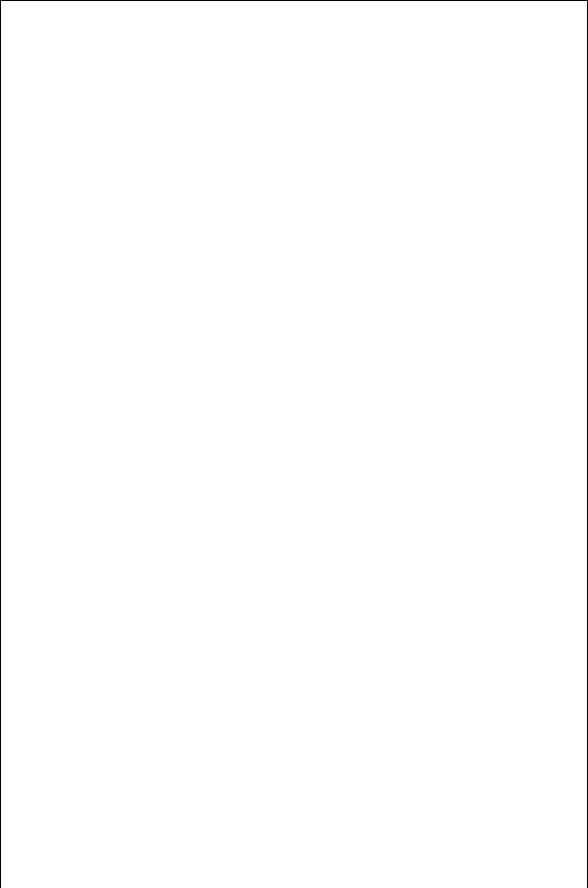
REFERENCES 31
PROBLEMS
2.1Calculate the resistance of a 1 m long copper wire over a frequency range of 100 MHz to 1 GHz when (a) the diameter of the wire is 31.2 mils (AWG #20), and (b) when it is 10.0 mils (AWG #30). Plot your results of ac resistance in terms of frequency.
2.2You are asked to determine the inductance of a solenoid when the form length is 1.5 in., the form diameter is 0.3 in., and there are 12 turns.
(a)What wire diameter would you choose?
(b)What is the inductance?
(c)What is the self-resonant frequency of the inductor?
REFERENCES
1.R. A. Pucel, “Design Considerations for Monolithic Microwave Circuits,” IEEE Trans. Microwave Theory Tech., Vol. MTT-29, pp. 513–534, 1981.
2.R. Williams, Gallium Arsenide Processing Techniques, Boston: Artech House, 1990.
3.P. E. Allen and D. R. Holberg, CMOS Analog Circuit Design, New York: Oxford University. Press, 1987, p. 66.
4.A. D. Moore, Fluid Mapper Patterns, Ann Arbor: Overbeck, 1956.
5.C. P. Lee, B. M. Welch, and R. Zucca, “Saturated Resistor Load for GaAs Integrated Circuits,” IEEE Trans. Microwave Theory Tech., Vol. MTT-30, pp. 1007–1013, 1982.
6.V. F. Perna, Jr. “A Guide to Judging Microwave, Capacitors,” Microwaves, Vol. 9, August, pp. 40–42, 1970.
7.V. F. Perna, Jr. “Chip Capacitor Dielectric Effects on Hybrid Microwave Amplifiers,”
Proc. International Society for Hybrid Microelectronics, October 13, 1971.
8.R. S. Muller and T. I. Kamins, Device Electronics for Integrated Circuits, 2nd ed., New York: Wiley, 1986, p. 190.
9.P. L. D. Abrie, The Design of Impedance-Matching Networks for Radio-Frequency and Microwave Amplifiers, Boston: Artech House, 1985.
10.R. G. Medhurst, “High Frequency Resistance and Capacity of Single-Layer Solenoids,” Wireless Engineer, March, p. 35, 1947.
11.P. R. Geffe, “The Design of Single-Layer Solenoids for RF Filters,” Microwave J., Vol 39, pp. 70–76, 1996.
12.H. M. Greenhouse, “Design of Planar Rectangular Microelectronic Inductors,” IEEE Trans. Parts, Hybrids, Packaging, Vol. 10, pp. 101–109, 1974.
13.M. Parisot, Y. Archambault, D. Pavlidis, and J. Magarshack, “Highly Accurate Design of Spiral Inductors for MMIC’s with Small Size and High Cut–off Frequency Characteristics,” 1984 IEEE MTT-S Digest, pp. 106–110, 1984.
14.N. M. Nguyen and R. G. Meyer, “Si IC-Compatible Inductors and LC Passive Filters,” IEEE J. Solid State Circuits, Vol. 25, pp. 1028–1031, 1999.
15.R. G. Arnold and D. J. Pedder, “Microwave Lines and Spiral Inductors in MCM-D Technology,” IEEE Trans. Components, Hybrids, Manufact. Tech., Vol. 15, pp. 1038– 1043, 1992.
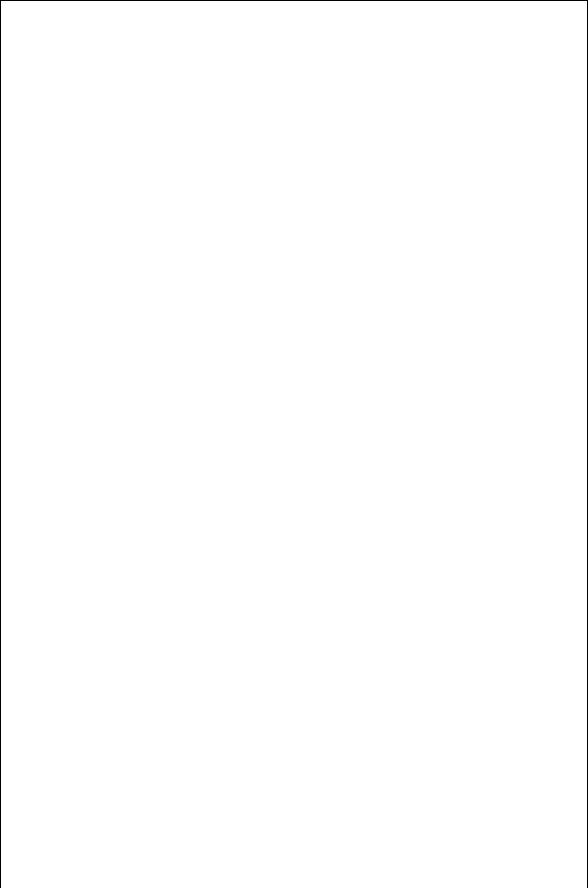
32 RESISTORS, CAPACITORS, AND INDUCTORS
16.S. Chaki, S. Andoh, Y. Sasaki, N. Tanino, and O. Ishihara, “Experimental Study on Spiral Inductors,” 1995 IEEE MTT-S Digest, pp. 753–756, 1995.
17.Z. Jiang, P. S. Excell, and Z. M. Hejazi, “Calculation of Distributed Capacitances of Spiral Resonators,” IEEE Trans. Microwave Theory Tech., Vol. 45, pp. 139–142, 1997.
18.A. M. Niknejad and R. G. Meyer, “Analysis, Design, and Optimization of Spiral Inductors and Transformers for Si RF IC’s,” IEEE J. of Solid State Circuits, Vol. 33, pp. 1470–1481, 1998.
19.E. Pettenpaul, H. Kapusta, A. Weisgerber, H. Mampe, J. Luginsland, and I. Wolff, “CAD Models of Lumped Elements on GaAs up to 18 GHz,” IEEE Trans. Microwave Theory Tech., Vol. MTT-36, pp. 294–304, 1988.
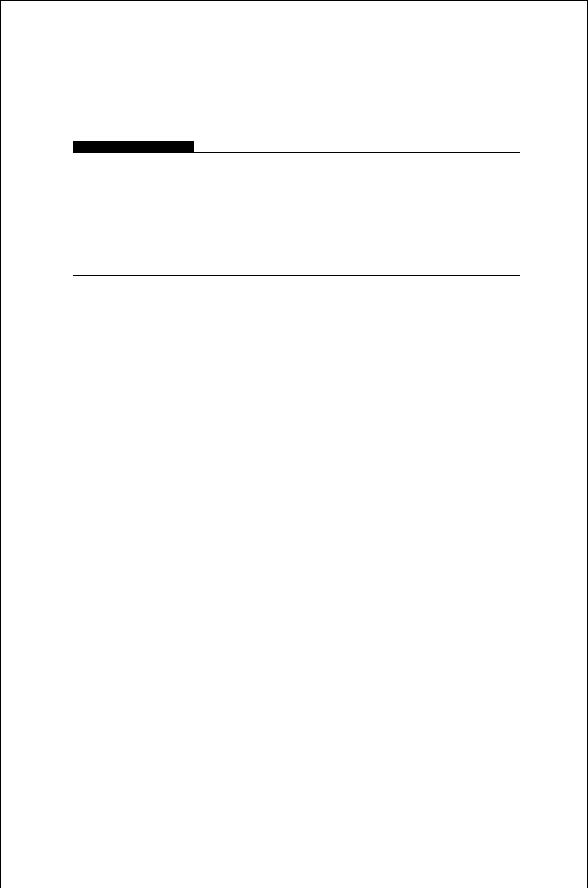
Radio Frequency Circuit Design. W. Alan Davis, Krishna Agarwal
Copyright 2001 John Wiley & Sons, Inc.
Print ISBN 0-471-35052-4 Electronic ISBN 0-471-20068-9
CHAPTER THREE
Impedance Matching
3.1INTRODUCTION
A major part of RF design is matching one part of a circuit to another to provide maximum power transfer between the two parts. Even antenna design can be thought of as matching free space to a transmitter or receiver. This chapter describes a few techniques that can be used to match between two real impedance levels. While some comments will be made relative to matching to a complex load, the emphasis will be on real impedance matching. The first part of this chapter will discuss the circuit quality factor, Q. The Q factor will be used with some of the subsequent matching circuit designs.
3.2THE Q FACTOR
The the circuit Q factor is defined as the ratio of stored to dissipated power in the following form:
2 Ð max instantaneous energy stored
Q D |
|
3.1 |
|
||
|
energy dissipative per cycle |
For a typical parallel RLC circuit, the Q becomes
ωC
Q D |
|
3.2 |
|
||
|
G |
where G is 1/R. For a series RLC circuit,
ωL
Q D |
|
3.3 |
|
||
|
R |
It should be emphasized that Q is defined at circuit resonance. If the circuit reactance is plotted as a function of frequency, the slope of the reactance at
33

34 IMPEDANCE MATCHING
X
High Q
Low Q
ω
FIGURE 3.1 Reactance slope related to Q.
resonance is a measure of Q (Fig. 3.1). This is explicitly given as
|
ω0 |
|
dB |
|
Q D |
|
|
|
3.4 |
|
|
2G dω ω0
where B is the susceptance and G the conductance. Alternately,
Q D |
ω0 |
|
dX |
3.5 |
|
2R dω |
|||||
|
ω0 |
where R and X are the resistance and reactance of the circuit. For a series RLC circuit this latter formula will result in the solution given by Eq. (3.3). On the other hand, the Q of a complicated circuit can be readily obtained from Eq. (3.4) or Eq. (3.5), even numerically if necessary.
3.3RESONANCE AND BANDWIDTH
The minimum insertion loss or maximum transmission of a parallel RLC circuit occurs at the resonant frequency of the circuit. When this circuit is excited by a current source, and the output is terminated with an open circuit, the transfer function is
Vout |
1 |
. |
3.6 |
|
|
D |
|
||
Iin |
1/R C jωC j/ωL |
This is shown in Fig. 3.2. The output voltage, Vout, drops from the resonant value p
by 2 (or 3 dB) because the denominator of the transfer function increases from
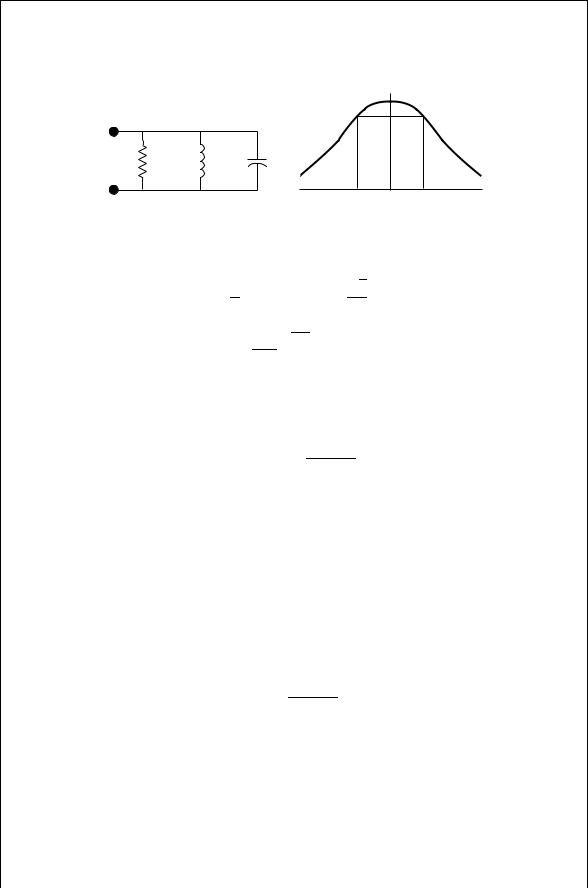
RESONANCE AND BANDWIDTH |
35 |
RL |
L |
C |
w1 w0 w2
FIGURE 3.2 Simple parallel resonant circuit.
1/R at resonance to |
p2 |
1 |
C jωC jωL D 3.7
R R
p
The resonant frequency is ωp0 D 1/ LC, and the value of Q given in Eq. (3.2) can also be written as Q D R C/L. Using these two facts, Eq. (3.7) becomes explicitly a quadratic equation in ω2:
ω4C2L2R2 ω2 2CLR2 C L2 C R2 D 0 |
3.8 |
The two solutions for ω2 are
ω2 D ω02 |
|
|
|
1 |
1 |
|
|
1 |
|
|
|
|
|
|
|
|
|
|
|||||||||||
1 C |
|
|
|
š |
|
|
|
|
|
1 C |
|
|
|
|
|
|
|
|
|
|
|
|
|||||||
2Q2 |
Q |
|
|
4Q2 |
|
|
|
|
|
|
|
|
|||||||||||||||||
|
|
|
|
|
|
|
|
|
|
|
|
|
|
|
|
|
|
|
|
|
|
|
|
|
|
|
|||
ω2 D ω02 |
|
|
|
1 |
|
1 |
|
|
|
|
1 |
|
|
|
1 |
|
|
|
|
|
|||||||||
1 C |
|
|
š |
|
|
|
|
1 C |
|
|
C |
|
|
|
|
|
|
|
|||||||||||
4Q2 |
Q |
4Q2 |
4Q2 |
|
|
|
|||||||||||||||||||||||
|
|
|
|
|
|
|
|
|
|
|
|
|
|
|
|
|
|
|
|
|
|
|
|
|
|
||||
ω2 D ω02 |
|
|
|
1 |
|
|
|
|
|
|
|
1 |
|
|
|
|
|
|
1 |
|
1 |
|
|||||||
|
1 C |
|
š |
|
Ð 1 C |
|
š |
|
3.9 |
||||||||||||||||||||
|
4Q2 |
2Q |
4Q2 |
2Q |
In this expression, 4R4/ω04 is replaced by 4L4Q4. This has been written as a product of two equal terms, so the original quartic equation has two pairs of equal roots. Taking the square root of Eq. (3.9) provides the two 3 dB frequencies of the resonant circuit:
1 |
1 |
|
||
ω1,2 D ω0 1 C |
|
š |
|
3.10 |
4Q2 |
2Q |
The 3 dB bandwidth of the resonant circuit is the difference between the two
3 dB frequencies: |
|
|
|
1 |
rad/s |
3.11 |
|
f D ω2 ω1 D |
|
||
RC |
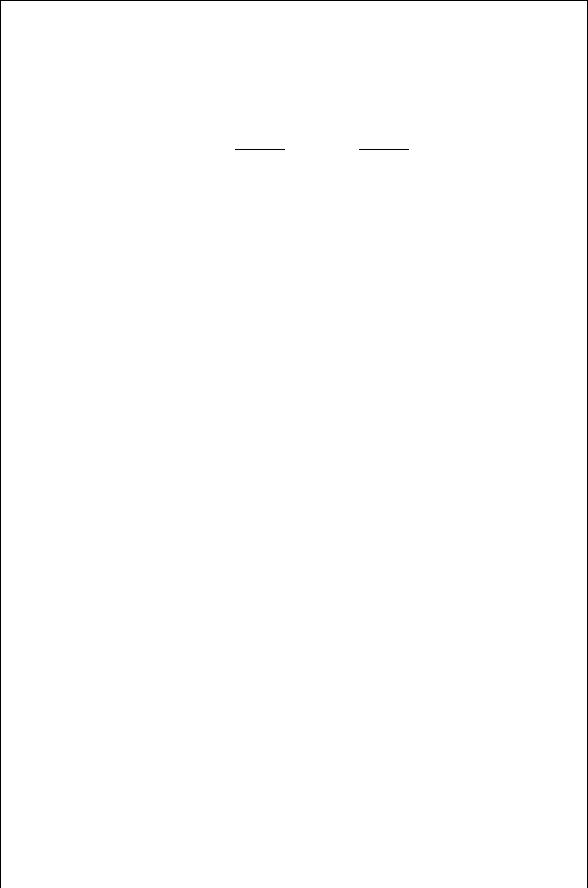
36 IMPEDANCE MATCHING
The response is clearly not symmetrical about the resonant frequency, ω0. The resonant frequency can be found by taking the geometric mean of the two solutions of Eq. (3.10) rather than the arithmetic mean:
ω1 Ð ω2 D ω02 |
1 |
1 |
1 |
1 |
|
||||||
1 C |
|
|
|
Ð 1 C |
|
C |
|
|
|||
4Q2 |
2Q |
4Q2 |
2Q |
||||||||
ω0 D p |
|
|
|
|
|
|
3.12 |
||||
ω1ω2 |
|
|
|
|
However, for narrow bandwidths, the arithmetic mean of the the two 3 dB frequencies can be used with a small error.
3.4UNLOADED Q
In real physical reactive elements there are always some resistive losses. The loss in a capacitor or an inductor can be described in terms of its Q. For example, if a lossy inductor is placed in parallel with a lossless capacitor, the Q of the resulting parallel circuit is said to be the circuit Q of the inductor. The inductor Qind then is
Qind D ω0CR D |
R |
3.13 |
ω0L |
||
or |
|
|
R D XLQind |
3.14 |
Similarly, for a lossy capacitor, its resistive component could be expressed in terms of the capacitor Qcap. If the inductor, capacitor, and a load resistance, RL are placed in parallel, then the total resistance is RT:
1 |
|
1 |
|
|
|
1 |
|
1 |
|
3.15 |
|||||||
|
|
|
D |
|
|
C |
|
|
|
C |
|
|
|
||||
|
RT |
RL |
QindXL |
QcapXC |
|||||||||||||
At resonance, XL D XC, so |
|
|
|
XL |
|
|
1 |
|
1 |
|
|
||||||
|
|
XL |
|
|
|
|
|
|
3.16 |
||||||||
|
|
|
D |
|
C |
|
|
C |
|
|
|||||||
|
|
RT |
RL |
|
Qind |
Qcap |
The unloaded Q, Qu is the Q associated with the reactive elements only (i.e., without the load). The bracketed term is the unloaded Q:
1 |
D |
1 |
C |
1 |
3.17 |
|
|
|
Qu Qind Qcap
3.5L CIRCUIT IMPEDANCE MATCHING
There are four possible configurations that provide impedance matching with only two reactive elements. In each case the design of the matching circuits is based
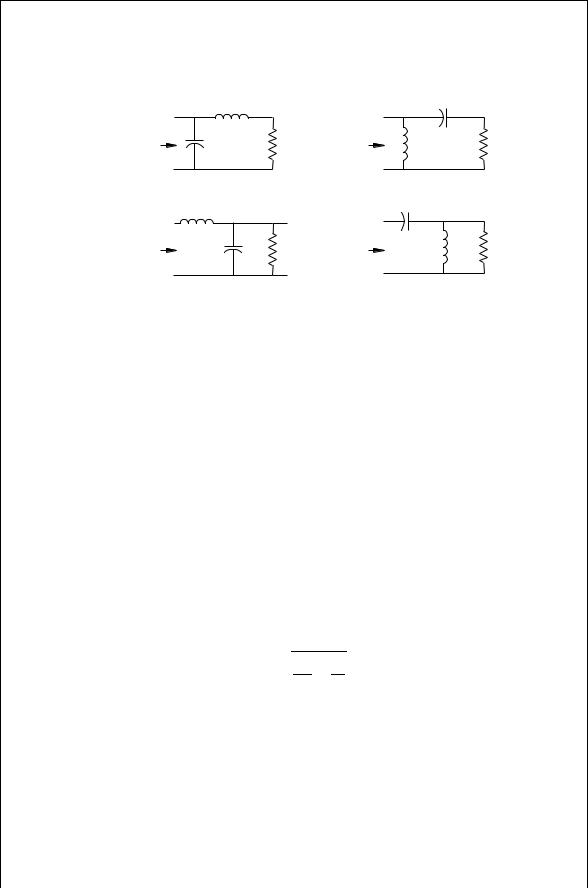
|
|
|
|
L CIRCUIT IMPEDANCE MATCHING |
37 |
|
|
|
L |
|
|
C |
|
|
|
|
|
|
|
|
Series |
R ' |
C |
R |
R ' |
L |
R |
|
|
(a) |
|
|
(b) |
|
|
|
L |
|
|
C |
|
|
|
|
|
|
|
|
Shunt |
R ' |
C |
R |
R ' |
L |
R |
|
|
(c) |
|
|
(d ) |
|
|
|
|
|
|
|
FIGURE 3.3 Four possible L matching circuits.
on the Q factor, a concept that will become even more important in designing broadband matching circuits [1]. Two of the circuits will be described as the series connection since the reactive element closest to the load resistance is a series reactance (Fig. 3.3a,b). The circuits with a shunt reactance closest to the load resistance are called the shunt connection (Fig. 3.3c,d). For the series connection in which the series reactance is an inductance, the total input admittance is given as follows:
Yin D jωC C |
|
1 |
|
|
|
|
|
||||
R |
C |
jωL |
|
||||||||
|
R |
|
|
|
ωL |
|
|||||
D |
|
|
C j ωC |
3.18 |
|||||||
|
|
|
|
||||||||
R2 C ωL 2 |
R2 C ωL 2 |
||||||||||
Resonance occurs when the total shunt susceptance, jB D 0. Thus |
|
||||||||||
|
C D |
|
|
L |
3.19 |
||||||
|
|
|
|||||||||
|
R2 C ω0L 2 |
Solution of this for the resonant frequency gives the following expression for the resonant frequency:
ω0 D |
1 R2 |
3.20 |
LC L2 |
The effect of the load resistor is to modify the resonant frequency somewhat. The conductive part of Yin at this frequency (where B D 0) can be found. Its
reciprocal is the input resistance, R0, of the circuit:
R0 |
D |
R2 C ω02L2 |
|
|
R |
|
|
|
D R 1 C Q12 |
3.21 |
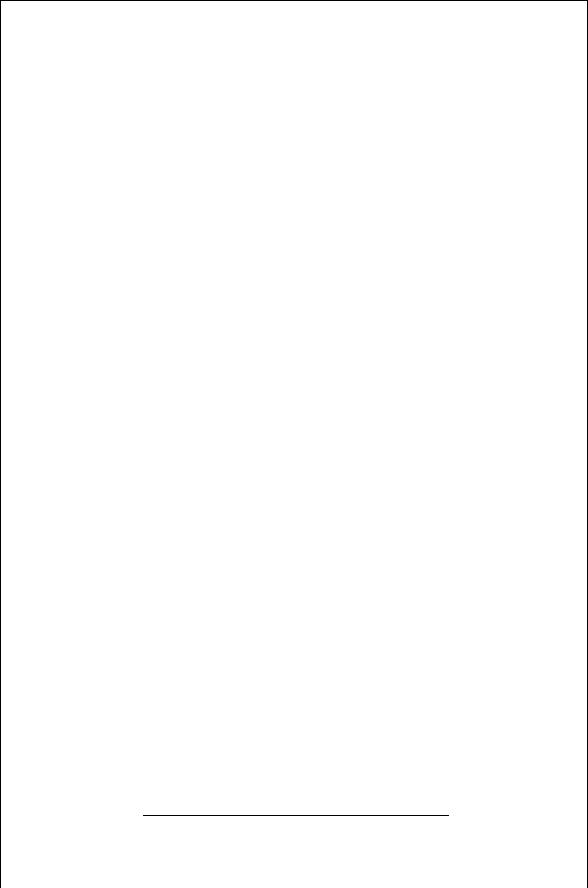
38 IMPEDANCE MATCHING
The subscript 1 for Q is present to emphasize this is a single resonator circuit. More complicated circuits might have several pertinent Q factors to consider.
At center frequency the reactance of the series part (i.e., not the capacitance part) will change with changing frequency. Its value can be found from the input admittance expression and is the amount the reactance changes because of the series inductance. This reactance change is
jX0 |
D |
jR2 C ω0L 2 |
3.22 |
|
ω0L |
||||
1 |
|
If X1 represents the series reactance, which in this case is ω0L, then the reactance of the series element can be found also in terms of Q:
jX10 |
1 |
|
|
D jX1 1 C |
|
3.23 |
|
Q2 |
|||
|
1 |
|
The second element in the LC section is chosen to resonate out this X01:
jX2 D jX10 |
1 |
|
||||
D jX1 1 C |
|
|
||||
Q2 |
||||||
|
|
|
1 |
|
||
D |
jR0 |
|
3.24 |
|||
Q1 |
||||||
|
|
|
In the typical synthesis problem, R0 and R are known. Equation (3.21) gives the necessary value of Q1, Eq. (3.3) gives the required L, and Eq. (3.24) gives the required C. This procedure is summarized in Table 3.1.
A similar procedure can be applied for the shunt connection in which the capacitance is closest to the load resistance. The input impedance is expressed as follows:
Zin D jωL C |
|
|
1 |
|
|
|
|
|
|
||
G |
C |
jωC |
|
|
|
|
|||||
|
G |
|
|
|
ωC |
|
|
||||
D |
|
|
C j ωL |
|
3.25 |
||||||
|
|
|
|||||||||
G2 C ωC 2 |
G2 C ωC 2 |
||||||||||
TABLE 3.1 |
L Matching Circuit Design Where X1, B1 are |
||||||||||
the Reactance or Susceptance Closest to the Load R |
|
|
|||||||||
|
|
|
|
|
|
|
|
|
|
||
Circuit |
R0 |
|
|
|
jX2 |
|
|
Q1 |
|
||
Series |
R 1 C Q12 |
|
|
jX1 1 C 1/Q12 |
X1/R |
||||||
|
2 |
|
|
or jR0/Q1 |
2 |
|
|
|
|||
Shunt |
R/ 1 C Q1 |
|
|
jX1/ 1 C 1/Q1 |
B1/G |
or jR0Q1
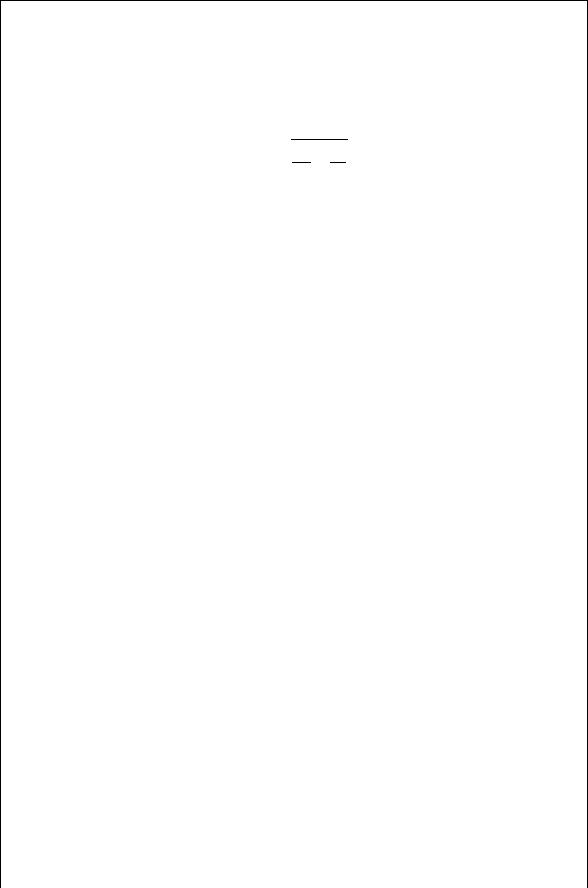
TRANSFORMATION CIRCUIT |
39 |
For resonance, the series reactance X D 0. Solution for the resonant frequency for the shunt connection is as follows:
ω0 D |
1 G2 |
3.26 |
LC C2 |
Substituting this back into the input impedance expression gives the input resistance:
R0 D |
|
|
|
1/G |
|
|
|
|
|
1 |
C |
ω C/G 2 |
|
||||||
|
|
0 |
|
|
|
|
|||
D |
|
R |
|
|
|
|
|
3.27 |
|
|
|
|
|
|
|
||||
1 C Q12 |
|
|
|
||||||
The reactance associated with the capacitance is |
|
||||||||
jX0 |
|
|
|
jω0C |
|
|
|||
|
|
|
|
|
|
||||
1 D G2 C ω0C 2 |
|
||||||||
Since jX1 D 1/jωC, |
|
|
|
|
|
|
|
|
|
jX10 D |
|
jX1 |
|
|
|
3.28 |
|||
|
|
|
|||||||
|
|
/Q2 |
|
||||||
|
|
|
1 C 1 |
1 |
|
|
|
||
|
D R0Q1 |
|
|
|
3.29 |
Since jX2 D jX1, the values in Table 3.1 are obtained.
The major feature that should be recognized, whether dealing with elaborate lumped circuits or microwave circuits, if the impedance level needs to be raised, a series connection is needed. If the impedance needs to be lowered, a shunt connection is needed. Furthermore, since the design is based on a resonance condition, the two reactances in the circuit must be of the opposite type. This means two inductors or two capacitors will not work.
3.6p TRANSFORMATION CIRCUIT
In the previous L matching circuit, the value for Q is completely determined by the transformation ratio. Consequently there is no independent control over the value of Q which is related to the circuit bandwidth. Addition of a third circuit element gives flexibility to design for bandwidth. If a design begins with a shunt L matching circuit, then addition of another shunt susceptance on the other side of the series element provides the necessary circuit flexibility to be able to choose the circuit Q as a design parameter. The resulting matching