
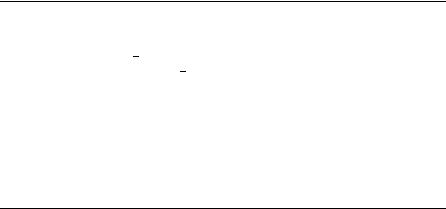
Supplement F2: The Chemistry of Amino, Nitroso, Nitro and Related Groups.
Edited by Saul Patai Copyright 1996 John Wiley & Sons, Ltd.
ISBN: 0-471-95171-4
CHAPTER 20
The synthesis and uses of isotopically labelled amino and quaternary ammonium salts
KENNETH C. WESTAWAY |
|
Department of Chemistry, Laurentian University, Sudbury, Ontario, |
|
Canada P3E 2C6 |
|
Fax: 705-675-4844; e-mail: KWESTAWA@NICKEL.LAURENTIAN.CA |
|
I. INTRODUCTION . . . . . . . . . . . . . . . . . . . . . . . . . . . . . . . . . . . . . . |
893 |
II. THE THEORY OF KINETIC ISOTOPE EFFECTS . . . . . . . . . . . . . . . . |
894 |
A. Heavy-atom Kinetic Isotope Effects . . . . . . . . . . . . . . . . . . . . . . . . |
894 |
B. Primary Hydrogen Deuterium Kinetic Isotope Effects . . . . . . . . . . . . |
895 |
C. Secondary Alpha Hydrogen Deuterium Kinetic Isotope Effects . . . . . . |
896 |
III.USING KINETIC ISOTOPE EFFECTS TO ELUCIDATE
THE MECHANISM OF BENZIDINE-TYPE REARRANGEMENTS
OF AMINES . . . . . . . . . . . . . . . . . . . . . . . . . . . . . . . . . . . . . . . . . |
897 |
IV. USING KINETIC ISOTOPE EFFECTS TO MODEL |
|
THE SN2 TRANSITION STATES FOR REACTIONS INVOLVING |
|
QUATERNARY AMMONIUM SALTS . . . . . . . . . . . . . . . . . . . . . . . . |
932 |
A. The Menshutkin Reaction . . . . . . . . . . . . . . . . . . . . . . . . . . . . . . . |
932 |
B. The SN2 Reactions of Quaternary Ammonium Salts . . . . . . . . . . . . . |
938 |
V. REFERENCES . . . . . . . . . . . . . . . . . . . . . . . . . . . . . . . . . . . . . . . . |
946 |
I. INTRODUCTION
This topic has been reviewed in ‘The Chemistry of the Functional Groups’ series published in 19821. It is not the purpose of this chapter to provide a complete literature review. Rather, this chapter will discuss some of the new methods of measuring and interpreting kinetic isotope effects that have been used to determine the mechanisms of the reactions of amines and amine derivatives.
893
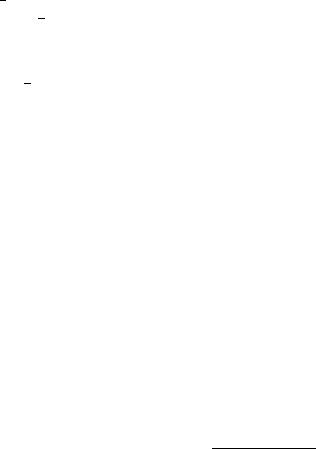
894 |
Kenneth C. Westaway |
II.THE THEORY OF KINETIC ISOTOPE EFFECTS
A.Heavy-atom Kinetic Isotope Effects
Several monographs2 5 have detailed discussions dealing with heavy-atom and primary and secondary hydrogen deuterium kinetic isotope effects. The monograph by Melander and Saunders5 covers the entire area particularly well. For this reason, only a brief summary of the theory of kinetic isotope effects as well as their important uses in the determination of reaction mechanism and transition-state geometry will be presented.
The Bigeleisen treatment6 8, based on Eyring and coworkers’ absolute rate theory9, assumes that there is a single potential energy surface along which the reaction takes place, and that there is a potential energy barrier separating the reactants from the products. The reaction occurs along the path over the lowest part of the barrier with the transition state at the top of the barrier, i.e. it lies at the energy maximum along the reaction coordinate but at an energy minimum in all other directions. The transition state is assumed to be in equilibrium with the reactants and products and to have all the properties of a stable molecule, except that one vibrational degree of freedom has been converted into motion along the reaction coordinate.
A C B ! |
|
Transition |
|
‡ |
|
state |
! Products |
1 |
The kinetic isotope effect for this reaction is:
k1 |
1 |
|
Q1‡ QA2 |
|
QB2 |
2 |
|||
|
D |
|
Ð |
|
Ð |
|
Ð |
|
|
k2 |
2 |
Q2‡ |
QA1 |
QB1 |
where the subscripts 1 and 2 refer to the molecules containing the lighter and heavier isotopes, respectively, and the Qs are the complete partition functions for reactants A and B. Setting 1 D 2 and applying the harmonic approximation to all nonlinear gas molecules leads to an expression for Q2/Q1 (equation 3), where S1 and S2 are the symmetry numbers of the respective molecules, the Ms are the molecular weights, the Is are the moments of inertia about the three principal axes of the n-atom molecules, and the s are the fundamental vibrational frequencies of the molecules in wave numbers.
Q2 |
|
S1 |
IA2IB2IC2 |
|
1/2 |
|
M2 |
|
3/2 |
3n 6 |
|
1i 2i hc |
|
1 |
exp hc 1i/kTN |
|
||
|
D |
|
|
|
|
|
|
|
|
|
|
|
|
|
|
|||
Q1 |
S2 |
IA1IB1IC1 |
|
|
M1 |
|
i |
2kT |
1 |
exp hc 2i/kT |
||||||||
|
|
|
|
|
|
|
N |
|
|
|
N |
|
||||||
|
|
|
|
|
|
|
|
|
|
|
|
|
|
|
|
|
|
3
Using various approximations, a solution to the isotopic rate ratio equation can be obtained. It is found that the isotope rate ratio, k1/k2, is dependent on the force constant changes which occur in going from the reactants to the transition state. Consequently, if C X bond rupture (where the isotopically labelled atom X can be halogen, sulfur, nitrogen, etc.) has not progressed at the transition state of the rate-determining step of the overall reaction, there is no change in the force constants involving the isotopic atom and the isotope rate ratio, k1/k2, will be equal to one. An isotope rate ratio greater than one will be observed if there is a decrease in the force constants at the transition state of the slow step. The greater the decrease in the force constant, the larger the magnitude of the isotope effect.
The observation of a heavy-atom isotope effect, therefore, allows one to determine whether C X bond weakening (a decrease in force constant) has occurred when the reactant is converted into the transition state of the rate-determining step. Calculations by
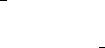
20. The synthesis and uses of amino and quaternary ammonium salts |
895 |
Saunders10 and by Sims and coworkers11 have shown that the magnitude of the leavinggroup heavy-atom isotope effect varies linearly with the extent of C X bond rupture in the transition state for concerted elimination reactions and for nucleophilic substitution reactions, respectively. Since the magnitude of the isotope effect is directly related to the amount of C X bond rupture in the transition state, these isotope effects provide detailed information about the structure of the transition state.
B. Primary Hydrogen Deuterium Kinetic Isotope Effects
Although the zero-point energy differences between the isotopic molecules’ vibrations are not the only contribution to the isotope effect, they are, however, often the dominant term. This is particularly true for hydrogen deuterium kinetic isotope effects where the zero-point energy difference is large, and also for large molecules where isotopic substitution does not effect the mass and moment-of-inertia term significantly. It is usual to assume that the stretching modes are the most important in determining these isotope effects. This is based on the two assumptions: (i) that the bending vibrations are generally of a lower frequency and therefore have smaller zero-point energy differences for isotopic molecules, and (ii) the bending motions in the transition state will be similar to those in the substrates.
Applying these approximations to the rupture of a single C H bond in a unimolecular process leads to equation 4,
kD |
D exp |
|
2kTN |
H D |
4 |
kH |
|
|
hc |
|
|
where H and D are the ground-state symmetric stretching frequencies for the C H and C D bonds, respectively. Substitution of the appropriate frequencies into equation 4 gives an isotope effect of approximately seven at 25 °C.
For reactions involving a proton transfer from one molecule to another, however, the situation is more complex. Westheimer12 and Melander2 independently pointed out that, because bond formation and bond breaking are occurring concurrently, new stretching vibrations in the transition state which are not present in the reactants must be considered.
They considered the reaction:
AH C B ! [A- - -H- - -B]‡ ! A C HB |
5 |
where [A- - -H- - -B] is a linear transition state. If this transition state is regarded as a linear molecule, there are two independent stretching vibrational modes which may be illustrated as follows:
? ! |
! |
A- - -H- - -B |
A- - -H- - -B |
Symmetric |
Antisymmetric |
Neither of these vibrations corresponds to stretching vibrations of AH or BH. The ‘antisymmetric’ vibrational mode represents translational motion in the transition state and has an imaginary force constant. The ‘symmetric’ transition-state vibration has a real force constant but the vibration may or may not involve motion of the central H(D) atom2,12,13. If the motion is truly symmetric, the central atom will be motionless in the vibration and the frequency of the vibration will not depend on the mass of this atom, i.e. the vibrational frequency will be the same for both isotopically substituted transition states. It is apparent that under such circumstances there will be no zero-point energy difference
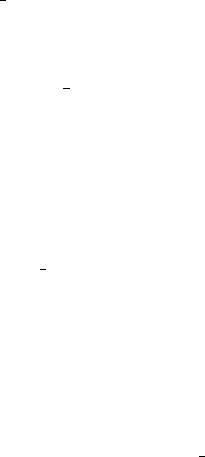
896 |
Kenneth C. Westaway |
between the deuteriumand hydrogen-substituted compounds for the symmetric vibration in the transition state. Hence, an isotope effect of seven at room temperature is expected since the difference in activation energy is the difference between the zero-point energies of the symmetric stretching vibrations of the initial states, i.e. 1/2 h H h D .
In instances where bond breaking and bond making at the transition state are not equal, bond breaking is either more or less advanced than bond formation, and the ‘symmetric’ vibration will not be truly symmetric. In these cases, the frequency will have some dependence on the mass of the central atom, there will be a zero-point energy difference for the vibrations of the isotopically substituted molecules at the transition state and kH/kD will have values smaller than seven.
It may be concluded that for reactions where the proton is less or more than one-half transferred in the transition state, i.e. the A H and H B force constants are unequal, the primary hydrogen deuterium kinetic isotope effect will be less than the maximum of seven. The maximum isotope effect will be observed only when the proton is exactly half-way between A and B in the transition state. This relationship is also found for carbon kinetic isotope effects where the isotopically labelled carbon is transferred between two atoms in the reaction10,11. This makes interpreting carbon isotope effects difficult.
C. Secondary Alpha Hydrogen Deuterium Kinetic Isotope Effects
In the preceding sections, the bond to the isotopic atom is broken or formed in the rate-determining step of the reaction. In these cases, the change in rate is referred to as a primary kinetic isotope effect. Isotopic substitution at other sites in the molecule has much smaller effects on the rate. These small isotope effects are collectively referred to as secondary kinetic isotope effects.
As with primary isotope effects, the origin of secondary isotope effects is considered to be mainly due to changes in force constants upon going from reactants to the transition state. For the most part, secondary isotope effects depend on the change in zero-point energy (ZPE). Smaller force constants for the isotopic nuclei in the transition state than in the reactant lead to an isotope effect greater than one (Figure 1a). When the force constants are greater in the transition state than in the reactant, on the other hand, an isotope effect of less than one is observed (Figure 1b).
Secondary alpha hydrogen deuterium kinetic isotope effects are determined when hydrogen is replaced by deuterium at the ˛- or reacting carbon. The generally accepted view originally proposed by Streitwieser and coworkers14 is that the alpha deuterium kinetic isotope effects are primarily determined by the changes in the out-of-plane bending vibrations in going from the reactants to the transition state. Solvolysis reactions proceeding via a carbocation are expected to give large normal isotope effects, kH/kD ˛. The maximum kH/kD ˛ expected per deuterium for various leaving groups are 1.22 for fluoride, 1.15 for chloride, 1.13 for bromide, 1.09 for iodide, 1.19 for ammonia and 1.22 for benzenesulfonate15,16.
Smaller alpha deuterium isotope effects are observed for reactions proceeding via the SN2 mechanism. This is due to steric interference by the leaving group and/or the incoming nucleophile with the out-of-plane bending vibrations of the C˛ H bonds. This leads to an increased force constant at the SN2 transition state, 1 (see Figure 1b), where Nu is the nucleophile and LG is the leaving group in the SN2 reaction.
In fact, small or inverse isotope effects, kH/kD ˛-D D 0.95 1.04, are observed for the SN2 reactions of primary substrates17. Recently, Wolfe and Kim18 pointed out that the changes in the stretching vibrations played the major role in determining the magnitude of these isotope effects. However, a more recent study by Poirier, Wang and Westaway19
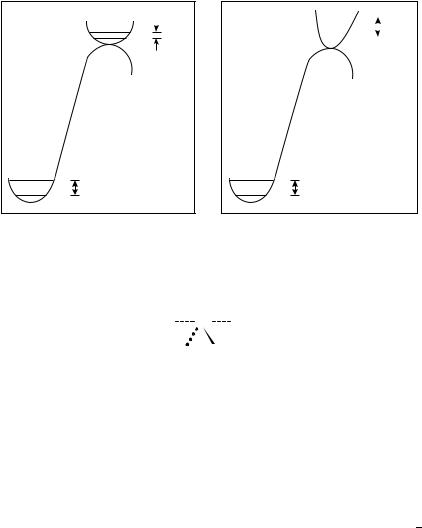
20. The synthesis and uses of amino and quaternary ammonium salts |
897 |
||||||||
H |
|
∆ZPE |
|
|
|
H |
|
|
∆ZPE |
|
|
|
|
|
|
||||
|
|
|
|
|
|
|
|||
|
|
|
|
|
|
|
|
||
|
|
|
|
|
|
|
|
|
|
D |
|
|
|
D |
|
||||
|
|
|
|
|
E |
|
E |
|
|
H |
∆ZPE |
H |
∆ZPE |
|
D |
D |
|||
|
|
|||
|
(a) |
|
(b) |
FIGURE 1. (a) A reaction where ZPE reactant is greater than ZPE transition state and kH/kD ˛ > 1.0.
(b) A reaction where ZPE reactant is less than ZPE transition state and kH/kD ˛ < 1.0
δ − |
|
δ + |
δ − |
Nu |
Cα |
LG |
(1)
showed that the magnitude of these isotope effects for reactions with a particular leaving group is determined by the nucleophile-leaving group distance in the SN2 transition state, i.e. the changes in the out-of-plane bending vibrations as the reactant is converted into the transition state.
III. USING KINETIC ISOTOPE EFFECTS TO ELUCIDATE THE MECHANISM OF
BENZIDINE-TYPE REARRANGEMENTS OF AMINES
Shine and coworkers have used heavy-atom kinetic isotope effects to determine the mechanism of several electrophilic aromatic substitution reactions. The particular reactions of interest are the collection of reactions known as ‘benzidine rearrangements’. The four mechanisms that have been proposed for these rearrangements are shown in equations 6 9 using the benzidine rearrangement of hydrazobenzene as the model. One possibility is the concerted rearrangement via polar transition states that was proposed by Ingold, Hughes and Banthorpe20 (equation 6). A second possibility proposed by Dewar21 is a nonconcerted reaction via -complexes (equation 7). A third possibility is that the reaction proceeds via a pair of radical cation intermediates within a solvent cage22 (equation 8) and the final possibility is a rate-determining proton transfer to the benzene ring (equation 9). It is not known whether the rearrangement is concerted with the addition of the ring proton or proceeds via several fast steps after the rate-determining proton transfer to the benzene ring. It is also not known whether the proton adds to the benzene ring bearing the protonated nitrogen22.
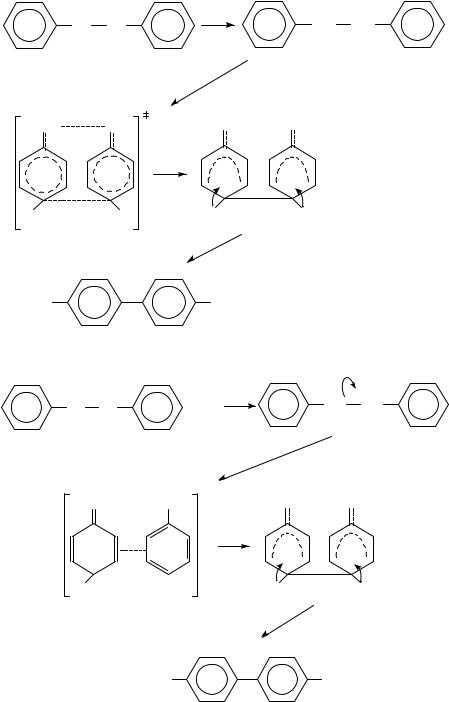
898 |
|
Kenneth C. Westaway |
|
|
NH |
NH |
2H + |
+ |
+ |
|
NH2 |
NH2 |
||
|
|
slow |
|
|
NH2 |
NH2 + 2 |
NH2 |
NH2 |
|
|
|
+ |
+ |
(6) |
|
|
|
||
H |
H |
H |
H |
|
|
|
− 2H + |
|
|
H2 N |
|
NH2 |
|
|
|
+ 2H+ |
+ |
+ |
|
NH NH |
NH2 |
NH2 |
|
|
|
|
slow |
|
|
NH2 |
NH2 |
NH2 |
NH2 |
|
|
|
+ |
+ |
(7) |
+ |
|
|
||
|
|
|
|
|
H |
|
H |
H |
|
π − complex |
|
−2H + |
|
|
|
|
|
|
H2 N |
NH2 |
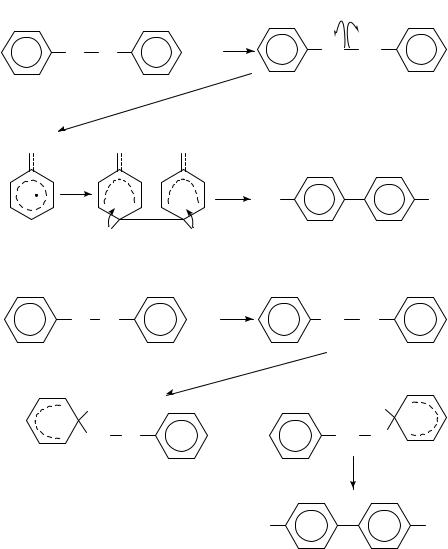
20. The synthesis and uses of amino and quaternary ammonium salts |
899 |
|||
|
+ 2H+ |
+ |
+ |
|
NH NH |
NH2 |
NH2 |
|
slow
NH2 |
NH2 |
NH2 |
|
|
+ |
+ |
+ |
− 2H |
+ |
|
|
|
|
H2 N |
H |
|
H |
|
|
NH NH |
+ H+ |
|
|
slow |
+ |
H |
|
+ |
and/or |
|
|
NH2 NH |
(8)
NH2
+
NH2 NH
H
+ |
+ |
|
|
NH2 NH |
|
−2H+ |
|
(9)
H2 N |
NH2 |
Shine and coworkers have used an extensive set of kinetic isotope effects to determine the mechanism of the benzidine rearrangement (equation 10). The major product of the rearrangement, 4,40 -diaminobiphenyl, or benzidine (2), accounts for 70% of the product, while 4,20 -diaminobiphenyl or diphenyline (3) accounts for the rest. The reaction is second order in sulfuric acid, so the diprotonated hydrazobenzene is obviously an intermediate in this reaction. A large nitrogen isotope effect of 1.022223,24 indicated that N N bond rupture occurred in the rate-determining step of the reaction. It is worth noting that these isotope effects were measured by comparing the isotopic composition of the products formed from mixtures of the unlabelled and the labelled compound containing two 15N isotopes per molecule, by whole-molecule isotope ratio mass spectrometry. This technique involves isolating the product from the reaction and analyzing it by mass spectrometry. In
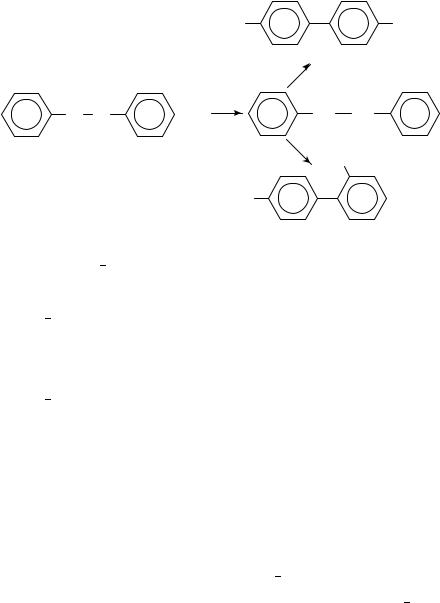
900 |
Kenneth C. Westaway |
these experiments, the isotopic ratio of the molecule (the ratio of the labelled to unlabelled molecules) was determined by measuring the isotopic composition of the molecule several thousand times. Typically, between 15,000 and 30,000 scans were used to determine the isotopic composition of the product. This very large number of scans was required because the heavy-atom isotope effects Shine and his coworkers measured were very small.
|
|
H2 N |
NH2 |
|
|
(2) |
|
|
+ 2H+ |
+ |
+ |
NH NH |
NH2 |
NH2 |
(10)
NH2
H2 N
(3)
Whole-molecule isotope ratio mass spectrometry was also used to measure the secondary hydrogen deuterium kinetic isotope effect of 0.962 found for the formation of benzidine from mixtures of the undeuterated and 4,40 -dideuterohydrazobenzene. This isotope effect illustrated that the bonding to these hydrogens was altered in the rate-determining step of the reaction. Model calculations indicated that the hydrogen deuterium isotope effect would have been normal if the reaction had proceeded via the -complex mechanism. Finally, a k12/k14 of 1.050 found for the formation of benzidine when the label was at C-4 of the hydrazobenzene, demonstrated that the C-4 carbon was also altered in the rate-determining step of the reaction. The nitrogen heavy-atom kinetic isotope effect of 1.0222 indicated that the N N bond is also breaking in the slow step of the reaction. Thus, finding a significant nitrogen, secondary hydrogen deuterium and carbon (C-4) kinetic isotope effect led the authors to conclude that the benzidene rearrangement of hydrazobenzene to benzidine occurs by the concerted mechanism shown in equation 6.
The nitrogen kinetic isotope effect of 1.063 for the formation of diphenyline confirmed that rupture of the N N bond was also involved in the rate-determining step of this reaction. However, the magnitude of the nitrogen isotope effect found in the formation of the diphenyline is very different from the isotope effect of 1.022 found for the formation of benzidine. This clearly indicates that this reaction proceeds via a very different transition state than that for the formation of benzidine and it was concluded that it probably occurred via the -complex mechanism (equation 7). This conclusion is supported by the very small (effectively zero) carbon isotope effects found for the formation of diphenyline22. In fact, a k12/k13 of 1.0000 was found by whole-molecule isotope ratio mass spectrometery and a k12/k14 of 1.0011 was obtained when a scintillation counter technique was used. This lack of a carbon isotope effect indicates clearly that carbon carbon bond formation does not occur in the rate-determining step of the reaction that forms diphenyline. Since N N bond rupture does occur in the slow step of the reaction to form diphenyline, but carbon carbon bond formation does not, it is obvious that diphenyline is not formed by a concerted
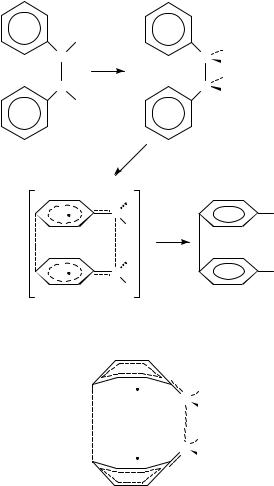
20. The synthesis and uses of amino and quaternary ammonium salts |
901 |
mechanism but must involve the formation of an intermediate, and it was concluded that it occurred via the -complex mechanism (equation 7). Further confirmation of the-complex mechanism was provided by the calculated isotope effects for the -complex mechanism. The calculated nitrogen kinetic isotope effect for the formation of diphenyline by the dissociative -complex mechanism was 1.055, in excellent agreement with the 1.063 found experimentally. A rationale for the different mechanisms for the formation of benzidine and diphenyline was presented. The formation of benzidine is concerted because it occurs via an allowed [5,5] suprafacial sigmatropic shift whereas the concerted formation of diphenyline would occur via a forbidden [3,5] suprafacial reaction.
The above results were interpreted in terms of the concerted mechanism shown in Scheme 1, although the authors state that one cannot determine whether the rings are bent (Figure 2) or planar in the transition state of the concerted reaction.
H |
+ |
H |
|
N |
|||
N |
H |
||
2H+ |
+ |
||
|
H |
||
N |
N |
H |
|
|
|
||
H |
|
|
|
|
slow |
|
H |
+ |
+ |
2 + |
|
N |
NH2 |
|
|
H |
−2H+ |
|
|
|
+ |
H |
|
N |
NH2 |
|
|
H |
|
SCHEME 1
+ |
H |
|
N |
|
H |
|
H |
+ |
N |
H |
FIGURE 2. The possible bent configuration of the benzene rings for the concerted formation of benzidine
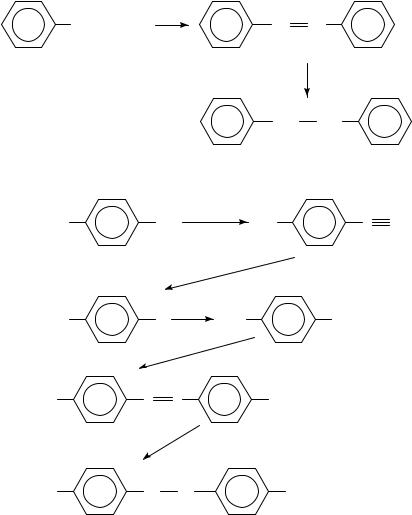
902 |
Kenneth C. Westaway |
The labelled substrates required for this study were synthesized as follows. The hydrazobenzene doubly labelled with nitrogen-15 was prepared from the commercially available 15N-labelled aniline (equation 11).
15 NH2 + MnO2 |
15 N 15 N |
|
∆ |
aq. acetone |
Zn, NH4 Cl |
(11) |
|
||
15 NH |
15 NH |
|
The 4,40-dideuteroazobenzene was prepared with 97% d2 by the sequence of reactions shown in Scheme 2.
O2 N |
|
HBF4 |
O2 N |
+ |
NH2 |
N N |
|||
|
|
NaNO2 , 0°C |
|
|
|
|
Cu2 O |
|
|
|
|
Na2 CO3 |
|
|
|
|
D3 PO2 |
|
|
O2 N |
|
Sn, HCl |
|
|
D |
H2 N |
|
D |
|
|
|
aq. MeOH |
|
|
|
|
Zn, NaOH |
|
|
D |
N |
N |
D |
|
aq. acetone
Zn, NH4 Cl
D |
NH NH |
D |
SCHEME 2
The 14C-labelled compound was prepared from the 14C nitrobenzene that was obtained by converting the commercially available 4-nitroaniline-1-14C into the diazonium tetrafluoroborate and reducing it with hypophospous acid (Scheme 3)25.
The 4,40 -13C2 azobenzene was prepared from the commercially available13C-labelled acetone by the sequence of reactions shown in Scheme 4.