
New Products and New Areas of Bioprocess Engineering
.pdf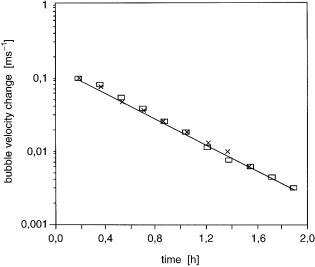
Recovery of Proteins and Microorganisms from Cultivation Media by Foam Flotation |
211 |
Fig. 4. Variations in the bubble velocity in recombinant E. coli culture at 13 and 16 h as a function of the time after the maximum velocity had been attained with SE9 AFA addition [51]
Animal cells are sensitive to direct bubble aeration, as several author have observed (e.g. [55, 56]). Pluronic F-68 (BASF, Ludwigshafen) is a copolymer of poly(oxyethylene) (POE) and poly(oxypropylene) (POP) with a relative molecular weight of about 8400, of which 80% is POE. In the molecule the hydrophobic POP moiety is flanked by two hydrophilic POE moieties. This surfactant have been widely used as a protective agent against hydrodynamic cell damage, because the direct cell–bubble interaction is eliminated. However, in medium supplemented with Pluronic F-68, the foaminess considerably increased. The highest foaming was observed in the middle of the exponential growth rate, with less foam being produced in the stationary and dying phases. To suppress the foam formation a silicone AFA (30% antifoam C emulsion; Sigma, USA) was used. Foam was completely eliminated in a medium supplemented with greater than 50 ppm AFA and 0.2% (w/v) Pluronic F-68. Relatively constant cell growth was found both in the bioreactor until 200 ppm AFA concentration; a marked reduction in cell growth was observed above that. Contrary to cell growth, relatively high monoclonal antibody (MAB) concentration was found in medium containing 200 ppm AFA. This enhancement effect on MAB concentration is attributed to the increased specific antibody production rate observed in the presence of Pluronic F-68 under lower cell growth. However, the cell damage by bubbles increased, probably because the AFA reduces the protective effect of Pluronic F68.
Van der Pol et al. [54] investigated the influence of a silicone AFA on the shear sensitivity of hybridoma cells in sparged cultures. They supplemented the medium with 1 g l–1 poly(ethylene glycol) PEG 6000 and PEG 20,000 to protect the cells from hydrodynamic damage. To suppress the foaming caused by the
212 |
K. Schügerl |
PEG, a poly(dimethylsiloxane) AFA (JT Baker, USA) was used, which increased the death rate.
3
Protein Flotation
Flotation has been used for centuries in the mining industry for the dressing and concentration of mineral ores and in waste water engineering. Despite many publications and several books on the use of flotation in the industry, only a few papers and short chapters in books have dealt with the application of flotation for protein and cell recovery [57, 58]. The theory of flotation has been discussed by Schulze [59] and Loewenberg and Davis [60]. Some biotechnological applications of flotation were presented in the handbook written by Rousseau [61].
The recovery of proteins from cultivation medium is usually performed by precipitation, adsorption, flocculation, extraction and ultrafiltration [62–64]. The adsorptive bubble separation techniques were considered in a book by Lemlich [65]. Foam flotation was described by Wilson and Clark [66]. However, in these books, no systematic investigations on the influence of equipment and operating parameters on protein enrichment and separation factors were published.
Foam flotation is especially suitable for protein recovery from aqueous solutions at low protein concentrations. BSA was used as a model protein in batch [67] and continuous operation [68–71]. b-Casein recovery [72] and binary mixtures (BSA/lysozyme, b-casein/lysozyme and b-casein/BSA) and their separation were also investigated [73].
The most common mode of operation of foam fractionation employed by various investigators is the single stage semibatch flotation: the protein solution used in batch mode and aerated continuously [71]. However, continuous multistage operation has the highest performance [70]. Therefore, this operation mode is discussed in detail.
3.1
Continuous Flotation of Proteins
Typical continuous laboratory flotation equipment has been described by Gehle and Schügerl [70], who investigated the recovery of BSA from aqueous solution by foam flotation. The thermostatted flotation setup consisted of a column with a 23-mm internal diameter, 49-cm bubbling liquid height and 30-cm foam layer height. Nitrogen gas saturated with water was distributed by perforated and porous plates, respectively. The protein solution was fed into the column at the height of the interface between the bubble column and the foam column. The interface was controlled by an overflow. The foam left the column at the top. The foam liquid was recovered by a mechanical foam breaker. The remaining liquid at the bottom was disposed of through the overflow (Fig. 5). Another thermostatted setup consisted of a column 25 mm in diameter, 44 cm bubbling liquid height and 18 cm foam layer height operated in the same way as the other

Recovery of Proteins and Microorganisms from Cultivation Media by Foam Flotation |
213 |
Fig. 5. Three-stage thermostatted flotation column (25 mm diameter). P feed; R liquid outlet with protein residue; S foam liquid outlet; N2 gas inlet; 1 glass frit; 2 thermostat; 3 perforated plates with overflow and down-comer; 4 liquid level in the three stages; 5 electrical conductivity probe; and 6 foam breaker (3000 rpm) [70]
214 |
K. Schügerl |
apparatus. This piece of equipment was operated as a threeand four-stage bubble column, respectively. The pH value of the feed solution was controlled by a pH meter.
The electrical conductivity of the foam was measured on-line to control the steady state of the process. Results were only accepted if they had been obtained under steady state conditions. The protein concentrations were determined by a photometer at 275.5 and 320 nm and the difference was used for to correct for the error due to the turbidity of the solution.
3.1.1
Characterization of Process Performance
The process performance was characterized by the protein enrichment (E), the protein separation (S), as well as by the protein recovery (R), i.e. the partition of protein between the foam liquid and residue liquid. Protein enrichment (E) is defined as:
CS |
protein concentration in foam liquid |
E = 410000092 |
|
CR |
protein concentration in feed |
protein separation (S) as:
CS |
protein concentration in foam liquid |
S = 410000096 |
|
CR |
protein concentration in residue liquid |
and protein recovery (R) as:
Mass of protein in the foam
R = 000006¥ 100(%)
Mass of protein in the initial feed
(6)
(7)
(8)
where CP is the protein concentration in the feed, CS the protein concentration in the foam liquid and CR the protein concentration in the residue liquid.
The results of protein analysis were controlled by the protein balance:
CPVtP = CSVtS + CRVtR |
(9) |
where VtP , VtS and VtR are the volumetric flow rates of the feed, the foam liquid and the residue liquid, respectively.
The foaminess S was determined according to Bikermann [74]. However, since the foaminess in a continuous flotation cannot be defined according to Eq. (1), the foam liquid volume flow VtS was used to characterize the foam property. This modified foaminess S* is approximately proportional to S defined according to Eq. (1) and for continuous operation is defined by Eq. (10):
VtS |
(10) |
S* = 41 |
|
Vtg |
|

Recovery of Proteins and Microorganisms from Cultivation Media by Foam Flotation |
215 |
Table 4. Examples of the performance of columns A, columns (single stage) B (four stage) and C (three stage), CP = 75 mg l–1 at 30 °C and pH 4.8 [70]
Column |
A |
B |
C |
|
|
|
|
S = CS /CR |
7.9 |
11.1 |
19.5 |
CS (mg l–1) |
237.5 |
282.3 |
870.0 |
CR (mg l–1) |
30.2 |
25.4 |
45.0 |
VtS (ml min–1) |
2.0 |
2.2 |
0.4 |
R (%BSA in S) |
66 |
80 |
43 |
Mean residence time t (min) |
19.3 |
18.4 |
n.d |
|
|
|
|
The recovery, i.e. the partition of protein between foam and residue, was also determined. Typical results are shown in Table 4. In the three-stage column the highest enrichment (E) was achieved, whereas in the four-stage column it was the best recovery (R) that was obtained. Under optimum operational conditions the performance of the protein recovery was considerably improved (Table 4).
BSA was also used as a model protein by Brown et al. [75] for protein recovery by foam flotation. They investigated the influence of feed concentration, superficial gas velocity, feed flow rate, bubble size, pH and ionic strength on the enrichment and recovery of BSA in a single-stage continuous flotation column.
3.1.2
Influence of Process Variables
The concentration of the protein in the foam liquid and in the residue depends on several parameters which interact in a complex way. It is difficult to develop a quantitative relationship between them for cultivation media. However, some qualitative interrelations can be recognized [70].
1.Parameters which increase the foam liquid volume flow,i.e. the water content in foam, diminish CS . Thus,VtS is increased and CS diminished by a reduction in:
–foam layer height (drainage is reduced)
–temperature (drainage is reduced)
–concentration of „structure breaker“ (NaClO4) and ethanol (above 1 vol%) (foaminess increased)
and by an increase in
–protein concentration in feed (foaminess increased)
–concentration of „structure maker“ (Na2SO4) and ethanol below 1 vol% (foaminess increased).
2.Some of the effects which increase the foam liquid volume flow diminish CR as well:
–concentration of ethanol above 1 vol% (solubility of protein decreased)
–aeration rate
–porosity of aerator (specific gas/liquid area is increased)
–concentration of „structure maker“ (Na2SO4) and ethanol below 1 vol%.

216 |
K. Schügerl |
3.When CS and CR change in the same direction, then:
–CS /CR increases if the aeration rate is reduced
–CS /CR diminishes if Na2SO4 or ethanol is added to the solution.
4.The separation (CS /CR) can be increased by a decrease in:
–aeration rate
–protein concentration in feed
–ethanol concentration above 1 vol%
and by an increase in
–porosity of the aerator
–temperature
–liquid and foam layer heights
–concentration of „structure breaker“ and ethanol concentration below 1 vol%, and
–at the isoelectric point (pH 4.8–5.0).
3.2
Separation of Protein Mixtures
Ostermaier and Dobiás [76] investigated the possibility of separating proteins with different isoelectric points (IEPs) by flotation from mixtures by varying the pH of the solution, together with certain concentrations of structure maker and structure breaker. They applied a mixture of the following proteins: fetuin, BSA, hemoglobin, myoglobin, chymotrypsinogen A and cytochrome c with IEPs in the range 3.5 to 10.2. Measurements of solutions of the same proteins at various pH values showed that the minimum surface tension occurs at the IEP of the protein concerned. The surface tension of the protein mixture as a function of the pH indicated that IEPs of particular proteins are nearly the same as the IEPs of the single proteins. Therefore, protein separation should be possible
Table 5. Performance of some protein flotations under optimum operational conditions
Protein |
pH |
T (°C) |
CP |
R(%) |
E(–) |
S(–) |
Ref. |
|
|
|
|
|
(mg l–1) |
|
|
|
|
|
|
|
|
|
|
|
|
|
BSA |
4.8 |
40 |
40 |
90 |
50 |
450 |
[70] |
|
b-casein |
5.3 |
25 |
20 |
62.4 |
54.7 |
181.3 |
[72] |
|
b-casein |
5.3 |
25 |
30 |
92.1 |
1.5 |
7.4 |
[72] |
|
Mixture of b-casein |
6.25 |
25 |
40 |
63.5 |
79.4 |
31.8a |
|
|
Lysozyme |
6.25 |
25 |
500 |
2.0 |
2.5 |
53.0b |
[73] |
|
Mixture of BSA |
4.6 |
25 |
10 |
41.9 |
74.2 |
|
||
Lysozyme |
4.6 |
25 |
500 |
0.9 |
1.4 |
|
[73] |
|
Mixture of b-casein |
5.3 |
25 |
40 |
49.4 |
23.5 |
0.6c |
|
|
BSA |
5.3 |
25 |
10 |
89.4 |
42.9 |
|
[73] |
|
|
|
|
|
|
|
|
|
|
a |
b-casein/lysozyme ratio. |
|
|
|
|
|
|
|
b |
BSA/lysozyme ratio. |
|
|
|
|
|
|
|
c |
b-casein/BSA ratio. |
|
|
|
|
|
|
|
Recovery of Proteins and Microorganisms from Cultivation Media by Foam Flotation |
217 |
by means of the surface tension vs. pH diagram. However, because of a strong overlapping of the protein peaks their separation was not possible.
Liu et al. [77] investigated the separation of BSA and hemoglobin by flotation in batch operation. An optimal separation factor a = S1(BSA)/S2 (HBB) = 14 was obtained at pH 3.9. Brown et al. [73] investigated the separation of binary mixtures of b-casein, lysozyme and BSA and obtained fairly good separations (Table 5).
The isolation and purification of human placental homogenate was investigated by Sarkar et al. [78] in a batch foam column. They found the optimum near to the IEP (pH 8.0). Column diameter and height were optimized. At a low gas flow rate the best separation from accompanying proteases was obtained.
3.3
Application of Additives
Miranda and Berglund [79] used a food grade polymer, (hydroxypropyl)methyl cellulose (HPMC), and ammonium sulfate as additives for the recovery of recombinant a-amylase by flotation. The enzyme was removed from the liquid phase by partition to a salted-out HPMC phase and the enzyme-containing polymer flocs were recovered by flotation. This system behaved in a manner similar to the flotation of mineral systems. The problem with this technique is the cost of the polymer and the separation of the enzyme from the polymer phase. Both of them complicate the process and increase the separation cost. In general, for protein recovery and separation, especially in the pharmaceutical industry, it is not proper to add chemicals to the feed, because they have to be removed from the product completely and this separation causes problems and additive costs.
3.4
Protein Denaturation
The application of foam flotation for the recovery of enzymes is often impaired by their denaturation and activity loss. By using nitrogen or carbon dioxide as sparging gas, respectively, instead of air, low volumetric flow rates (0.79 cm s–1) and operating at 16 °C and pH 3, denaturation can be suppressed. In the case of catalase the loss of enzyme activity was reduced to 0% and in the case of trypsin to 10% [80].
Varley and Ball [81] investigated the activities of lysozyme, pepsin and trypsin during foam separation. They found that with increasing protein concentration the enzyme activity loss diminished. According to Graham and Phillips [82, 83] the surface concentration of a protein at the gas/liquid interface changes with its bulk concentration. At higher bulk concentrations a multiprotein layer with high surface coverage is formed. A bubble surface covered by a multi-layer obviously reduces the residence time of the protein in the foam phase and protects the enzymes from denaturation. With increasing flow rate, the activity loss was reduced. 99% of the initial activity of lysozyme, 92% of
218 |
K. Schügerl |
pepsin and 90% of trypsin were retained at |
high flow rates, above |
300 cm3 min–1, at 2.2 mg ml–1 lysozyme, 0.2 mg ml–1 |
pepsin and 0.1 mg ml–1 |
trypsin initial concentrations [81]. |
|
3.5
Mathematical Modeling
To describe and quantify the effects of the various operational variables on the performance of foam flotation, several authors have developed mathematical models. Earlier investigators assumed equal-sized bubbles, infinite surface viscosity and accounted only for the gravity drainage [84–86]. Later on, the influence of the surface viscosity on the liquid drainage was also considered [87–89] and the liquid holdup profile in a foam column was predicted by considering the liquid drainage from the Plateau border [90]. The change in the bubble size distribution due to inter-bubble gas diffusion was also taken into account [91–94]. Uraizee and Narsimhan [95, 96] developed an advanced model for continuous foam concentration of protein which accounts for (i) kinetics of the adsorption of proteins in the liquid pool and in the foam, (ii) the liquid drainage from this film due to Plateau border suction, (iii) the gravity drainage of liquid from the Plateau border, and (iv) the bubble coalescence in the foam. The mass balances of liquid in the film and Plateau border, the protein balances in the film and Plateau border, and the balance of the bubbles are used with appropriate boundary conditions. The protein concentration in the liquid pool, the kinetics of adsorption of protein, the time of bubble formation, the residence time of the bubbles in the liquid pool and the coalescence rate are needed for the calculation.
In order to solve the balance equations, the velocities of the film and Plateau border drainage as well as the kinetics of the protein adsorption in the films are used. The velocity of drainage of Plateau borders, u, depends on the flow due to gravity and due to the gradient of Plateau border suction. By neglecting the
latter one obtains: |
|
Cu ap Çg |
(11) |
u ≈ 03 |
|
61 |
|
20 ÷3mP |
|
where Cv is the velocity coefficient for gravity drainage of the Plateau border, aP is the cross-sectional area of the Plateau border (m2), Ç is the density of the protein solution, g is the acceleration of gravity, and mP is the viscosity of the protein solution.
Since the velocity of the film drainage is small, protein adsorption in films is assumed to be diffusion controlled. The enrichment was calculated by using the protein concentration in the feed for its pool concentration. The surface concentration of a protein at the foam/liquid interface was calculated by the following equation:
qp |
dG |
dt at z = 0 |
(12) |
G = Gformation + ∫ |
5 |
||
0 |
dt |
pool |
|
Recovery of Proteins and Microorganisms from Cultivation Media by Foam Flotation |
219 |
where G is the surface concentration of protein, qP the residence time of the bubble in the liquid pool, Gformation the protein concentration at the end of the
bubble formation, 5dG the rate of protein adsorption onto the bubble during
dt pool
its travel through the liquid pool, and z is the axial distance from the foam/ liquid interface (m).
The film thickness and area of the Plateau border at the foam/liquid interface were obtained from the knowledge of the gas velocity and bubble size. The coupled partial differential equations of the balances were solved using a differential equation solving package. The surface concentration of the protein at the film interface is calculated by assuming a diffusion-controlled adsorption rate. From the mass balance the bottom flow rate and the liquid pool concentration were evaluated. Using these data the enrichment and recovery were calculated. This model predicts the enhancement of protein enrichment with increasing liquid pool height, decreasing gas velocities, increasing bubble size due to coalescence, high feed flow rates and low feed concentrations in good agreement with the measurements with BSA solution. The extension and application of this mathematical model for complex cultivation media is still to be achieved.
4
Flotation of Microorganisms
Centrifugal separators are applied in industry for the recovery of microbial cells from cultivation media. In waste water engineering a combination of flocculation and sedimentation is practiced. In the laboratory, cross-flow membrane separation is often used for retention of the cells. Some microorganisms and cells are enriched in the foam; therefore, flotation is suited for the recovery of particular microbial cells from cultivation medium.
Dognon and Dumonte [97] and Dognon [98] were the first to observe the enrichment of microorganisms in foam. To enhance the separation quaternary ammonium ions [99–101] or flocculants [102] were applied. Cell recovery without additives (collectors) was seldom used [103–107].All of these investigations were performed in batch operation.
Several investigations were carried out to remove toxic heavy metal ions from waste water by biosorption. Microbial cells loaded with heavy metals were recovered by flotation, e.g. Streptomyces griseus and S clavuligerus loaded with Pb [108] and Streptomyces pilosus loaded with Cd [109]. In these flotation processes the microbial cells were dead; therefore, they are not considered here. The removal of pyritic sulfur from coal slurries such as coal/water mixtures by Thiobacillus ferrooxidans and recovery of this iron-oxidizing bacterium by flotation is a special technique in the presence of high concentrations of solid particles (see e.g. [110]). The flotation of colloid gas aphrons was used for the recovery of yeast in continuous operation [111] for the recovery of micro algae, and in the presence of flocculants in batch operation [112]. These special techniques are not discussed here.
220 |
K. Schügerl |
4.1
Flotation of Yeast Cells
Systematic investigations of microbial cell recovery by foam flotation were performed by Hansenula polymorpha [113–117] and Saccharomyces cerevisiae
[118–123] in continuous operation. The equipment used for flotation was often identical to that used for protein flotation. The microorganisms were cultivated in laboratory reactors on synthetic media in the absence of antifoam agents in continuous operation and the cell-containing cultivation medium was collected in a buffer storage and was fed into the middle of the column, at the top of the interface between the bubble and the foam layers. The height of the interface was controlled by an overflow. The foam left the column at the top. The cells were recovered from the foam liquid by a mechanical foam destroyer. The liquid residue left the column through an overflow [113] (Fig. 6).
Hansenula polymorpha was cultivated in synthetic medium in the absence of an antifoam agent in stirred tank reactors (B20; B Braun, Melsungen and LF 14; Chemap), as well as in the 45-l tower loop reactor described by Buchholz et al. [124]. The foam was controlled by a mechanical foam destroyer (Fundafoam; Chemap) and by a destroyer constructed and built at the Institute of Technical Chemistry, University of Hannover, respectively. The influence of substrate type (glucose, ethanol and methanol) and concentrations of substrates and cells, the growth limiting component (O2 , P, and N), pH value, temperature, and presence of flocculation agent (CaCl2 ) on the process performance was investigated.
4.1.1
Characterization of Process Performance
The cell recovery process was characterized by the cell enrichment factor E*, cell separation factor S* and cell recovery factor R* :
|
CS* |
cell concentration in foam liquid |
|
E* = 41 |
= 000004 |
||
|
CP* |
|
cell concentration in medium |
S* |
CS* |
|
cell concentration in foam liquid |
= 41 |
= 000008 |
||
|
CR* |
cell concentration in residue liquid |
|
|
C* V* |
cell mass flow in foam |
|
|
S |
tS |
= 00002 |
R* = 0 |
|||
|
C* V* |
cell mass flow in medium |
|
|
R |
tP |
|
The cell mass balance |
|||
C*V* = C* V* + C* V* |
|||
P |
tS |
S |
tS R tR |
(13)
(14)
(15)
(16)
was used to control the accuracy of the cell mass analysis.
In Eqs. (10–12), CP* , CS* and CR* are the cell concentrations in the feed, the foam liquid and the residue liquid, and VtP*, VtS* and VtR* are the flow rates of the