
Garrett R.H., Grisham C.M. - Biochemistry (1999)(2nd ed.)(en)
.pdf



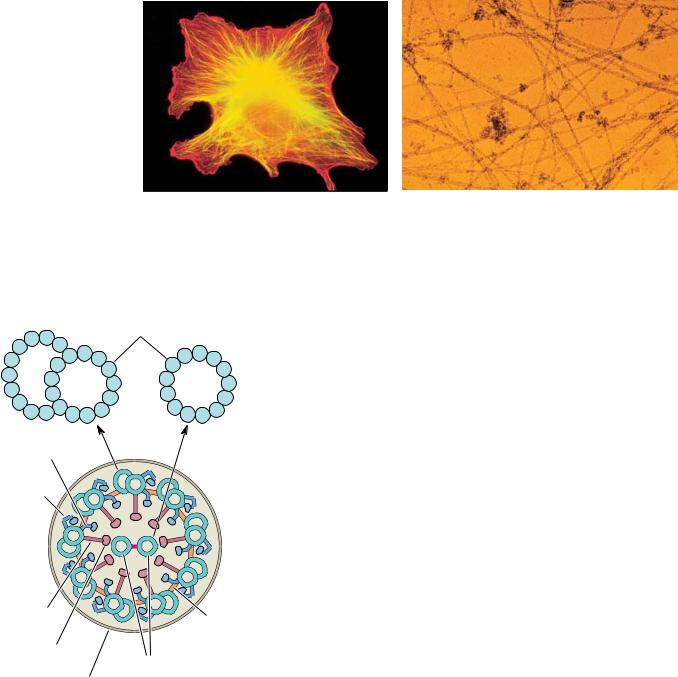
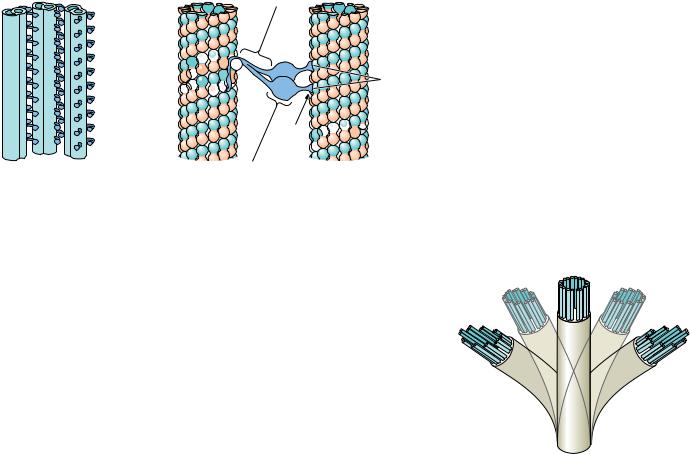
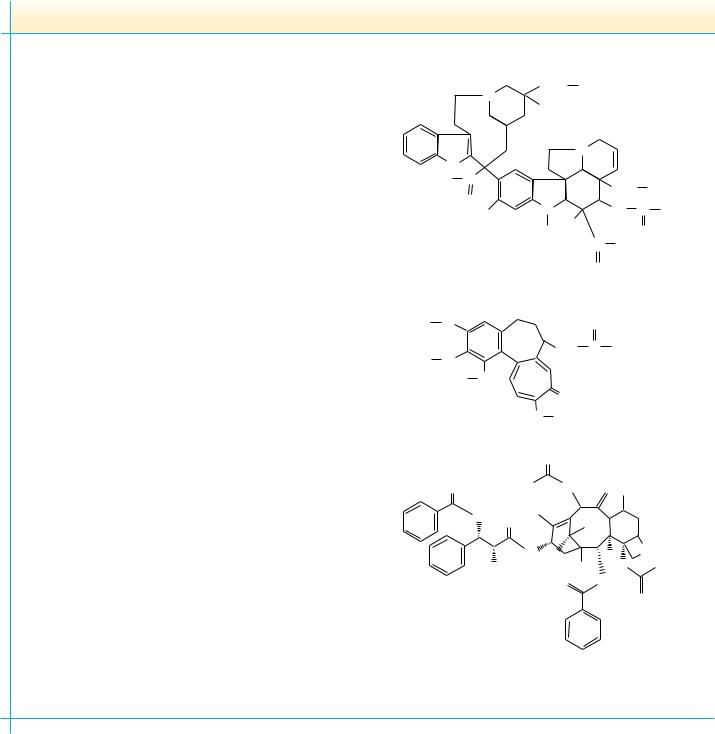

17.2 ● Microtubules and Their Motors |
539 |
(a)
Rough endoplasmic reticulum
Cell body |
|
|
Multivesicular |
Lysosome |
body |
|
Microtubule |
Nucleus
Vesicles |
Synaptic |
|
|
Golgi apparatus |
terminal |
|
|
Mitochondrion |
|
(b) |
Kinesin |
|
|
|
|
||
Organelle |
Vesicle |
|
|
Minus end |
Plus end |
FIGURE 17.8 ● (a) Rapid axonal transport |
|
along microtubules permits the exchange of |
|||
|
|
||
|
|
material between the synaptic terminal and the |
|
|
|
body of the nerve cell. (b) Vesicles, multivesicu- |
|
|
|
lar bodies, and mitochondria are carried |
|
|
|
through the axon by this mechanism. |
|
|
|
(Adapted from a drawing by Ronald Vale) |
kinesins, on the other hand, assist the movement of organelles and vesicles from the minus end to the plus end of microtubules, resulting in outward movement of organelles and vesicles. Kinesin is similar to cytosolic dyneins but smaller in size (360 kD), and contains subunits of 110 kD and 65 to 70 kD. Its length is 100 nm. Like dyneins, kinesins possess ATPase activity in their globular heads, and it is the free energy of ATP hydrolysis that drives the movement of vesicles along the microtubules.
The N-terminal domain of the kinesin heavy chain (38 kD, approximately 340 residues) contains the ATPand microtubule-binding sites and is the domain responsible for movement. Electron microscopy and image analysis of tubulin–kinesin complexes reveals (Figure 17.9) that the kinesin head domain is compact and primarily contacts a single tubulin subunit on a microtubule surface, inducing a conformational change in the tubulin subunit. Optical trapping experiments (see page 554) demonstrate that kinesin heads move in 8-nm (80-Å) steps along the long axis of a microtubule. Kenneth Johnson and his coworkers have shown that the ability of a single kinesin tetramer to move unidirectionally for long distances on a microtubule depends upon cooperative interactions between the two mechanochemical head domains of the protein.
