
- •PREFACE
- •CONTRIBUTORS
- •Abbott, David C.
- •Abelian Higgs model
- •Abelian string
- •aberration of stellar light
- •Abney’s law of additivity
- •A-boundary
- •absolute humidity
- •absolute magnitude
- •absolute viscosity
- •absolute zero
- •absorbance
- •absorptance
- •absorption cross-section
- •absorption fading
- •absorption line
- •abstract index notation
- •abyssal circulation
- •abyssal plain
- •acceleration
- •acceleration due to gravity (g)
- •accreted terrain
- •accretion
- •accretionary prism (accretionary wedge)
- •accretion disk
- •accretion, Eddington
- •accretion, hypercritical
- •accretion, Super-Eddington
- •Achilles
- •achondrite
- •achromatic objective
- •achronal set
- •acoustic tomography
- •actinides
- •action
- •activation entropy
- •activation volume
- •active continental margin
- •active fault
- •active front
- •active galactic nuclei (AGN)
- •active margins
- •active region
- •adiabat
- •adiabatic atmosphere
- •adiabatic condensation point
- •adiabatic cooling
- •adiabatic deceleration
- •adiabatic dislocation
- •adiabatic equilibrium
- •adiabatic index
- •adiabatic invariant
- •adiabatic lapse rate
- •ADM form of the Einstein–Hilbert action
- •ADM mass
- •Adrastea
- •advance of the perihelion
- •advection
- •advection dominated accretion disks
- •advective heat transfer (or advective heat transport)
- •aeolian
- •aerosol
- •aesthenosphere
- •African waves
- •afternoon cloud (Mars)
- •aftershocks
- •agonic line
- •airfoil probe
- •airglow
- •Airy compensation
- •Airy isostasy
- •Airy phase
- •Airy wave theory
- •Aitken, John
- •Aitken nucleus count
- •Alba Patera
- •albedo
- •albedo neutrons
- •albedo of a surface
- •albedo of single scattering
- •Alcyone
- •Aldebaran
- •Alfvénicity
- •Alfvén layer
- •Alfvén shock
- •Alfvén speed
- •Alfvén’s theorem
- •Alfvén wave
- •Algol system
- •Allan Hills meteorite
- •allowed orbits
- •all sky camera
- •alluvial
- •alluvial fan
- •alongshore sediment transport
- •Alpha Centauri
- •alpha particle
- •Altair
- •alternate depths
- •altitude
- •Amalthea
- •Amazonian
- •Ambartsumian, Viktor Amazaspovich
- •Amor asteroid
- •ampere
- •Ampere’s law
- •amphidrome (amphidromic point)
- •Am star
- •anabatic wind
- •analemma
- •Ananke
- •Andromeda galaxy
- •anelastic deformation
- •anemometer
- •angle of repose
- •Ångström (Å)
- •angular diameter distance
- •angular momentum L
- •angular velocity (
- •anisotropic
- •anisotropic scattering
- •anisotropic turbulence
- •anisotropic universe
- •anisotropy
- •annular eclipse
- •anomalistic month
- •anomalistic year
- •anomalous resistivity
- •anomaly
- •anomaly, South Atlantic
- •anorthosite
- •anoxia
- •Antarctic circle
- •antarctic circumpolar current
- •Antarctic ozone depletion
- •Antarctic ozone hole
- •Antarctic Zone
- •Antares
- •anthropic principle
- •anticyclone
- •anticyclonic
- •antidune
- •antinode
- •antiparticle
- •apastron
- •aperture correction
- •aperture synthesis
- •aphelion
- •aphotic zone
- •Ap index
- •apoapsis
- •Apollo asteroid
- •apparent horizon
- •apparent magnitude
- •apparent optical property (AOP)
- •apparent solar time
- •apse
- •Ap star
- •aquifer
- •aquitard
- •Arago point
- •arcade
- •archaeoastronomy
- •archaeomagnetism
- •Archean
- •Archimedes’ principle
- •Archimedian spiral
- •arc minute
- •arc second
- •Arctic circle
- •arctic oscillation (AO)
- •Arcturus
- •argon
- •argument of periapse
- •Ariel
- •array seismic observation
- •arrow of time
- •ascending node
- •aseismic front
- •aseismic region
- •asperity
- •association
- •asterism
- •asteroid
- •Asterope
- •asthenosphere
- •Astraea
- •astrochemistry
- •astrometric binary
- •astrometry
- •astronomical latitude
- •astronomical refraction
- •astronomical scintillation
- •astronomical tide
- •astronomical twilight
- •astronomical unit (AU)
- •astronomy, infrared
- •astronomy, ultraviolet: interstellar
- •asymmetry factor
- •asymmetry parameter
- •asymptotic
- •asymptotically simple space-time
- •asymptotic giant branch (AGB) star
- •asymptotic regime
- •Aten asteroid
- •Atlas
- •atmosphere
- •atmosphere effect
- •atmospheric angular momentum
- •atmospheric conductivity
- •atmospheric noise
- •atmospheric pressure
- •atmospherics
- •atmospheric tide
- •atomic mass
- •atomic number
- •atomic structure calculations — one-electron models
- •atomic time
- •aulacogen
- •aurora
- •aurora australis
- •aurora borealis
- •auroral cavity
- •auroral electrojet
- •auroral oval
- •auroral zone
- •autumnal equinox
- •available potential energy
- •avalanche
- •average cosine
- •averaging
- •Avogadro’s number
- •away polarity
- •AXAF
- •axial dipole principle
- •axionic string
- •azimuth
- •Baade–Wesselink method
- •Babinet point
- •baby universe
- •backarc spreading
- •back scattering
- •backscattering fraction
- •backshore
- •Backus effect
- •Baily’s beads
- •Ballerina model
- •Balmer series
- •banner cloud
- •bar detectors
- •barium release
- •Barnard’s star
- •baroclinic atmosphere or ocean
- •baroclinic instability
- •baroclinic wave
- •barotropic atmosphere or ocean
- •barotropic instability
- •barotropy
- •barred galaxies
- •barrier island
- •barriers
- •Barycentric Dynamical Time (TDB)
- •baryogenesis
- •basalt
- •basaltic lava
- •basement
- •basic MUF
- •Batchelor scale
- •Batchelor spectrum
- •Batchelor wavenumber
- •batholith
- •bathymetry
- •b-boundary
- •beach cusps
- •beachface
- •beach mining
- •beach morphology
- •beach nourishment
- •beach ridge
- •beams, ion
- •beam spread function
- •beam transmissometer
- •Beaufort wind scale
- •bed load
- •Beer’s law
- •Belinda
- •Benard cell
- •Benioff zone
- •benthic
- •benthos
- •Bergen school
- •Bergeron, Tor
- •berm
- •Bernoulli equation
- •Besselian year
- •beta decay
- •beta-effect
- •Beaufort Wind Scale Limits of Wind Speed at 10 m
- •beta-plane approximation
- •Bianca
- •biased vacuum states (domain wall)
- •bias frame, bias overscan
- •biasing parameter
- •Big Bang
- •Big Bang cosmology
- •Big Bang horizon problem
- •Big Bang nucleosynthesis
- •big blue bump
- •binary black holes
- •binary fraction
- •binary pulsar
- •binary star
- •binary star system
- •bioluminescence
- •biomass
- •biosphere
- •BIPM
- •Birkeland current
- •Birkhoff theorem (1923)
- •Bjerknes
- •Bjerknes circulation theorem
- •Bjerknes feedback
- •black aurora
- •black-body radiation
- •blackbody temperature
- •black frost
- •black hole
- •black hole binary
- •black hole horizon
- •black ice
- •blast wave shock
- •blazar
- •blizzard
- •BL Lacertae
- •BL Lacertae object
- •blocking
- •blocking patterns
- •blue clearing (Mars)
- •blue ice
- •blue jet
- •blue straggler
- •body waves
- •Bogomol’nyi bound
- •Bohr’s theory of atomic structure
- •bolide
- •bolometric correction (B.C.)
- •bolometric magnitude/luminosity
- •Boltzmann’s constant (
- •Bondi mass
- •Bonnor symmetry
- •bore
- •borrow site
- •boson
- •boson star
- •Bouguer correction
- •Bouguer gravity anomaly
- •bounce motion
- •boundary conditions
- •boundary layer pumping
- •Boussinesq approximation
- •Boussinesq assumption
- •Boussinesq equation
- •Bowen’s ratio
- •Boyle’s law
- •Brackett series
- •Bragg angle
- •Bragg Crystal Spectrometer (BCS)
- •braided river
- •Brans–Dicke theory
- •Brazil current
- •breaker zone
- •breakwater
- •breccias
- •Bremsstrahlung
- •bremstrahlung [thermal]
- •Brewster point
- •brightness
- •brightness temperature
- •bright point
- •brittle behavior (brittle fracture)
- •brittle-ductile transition
- •brittle-plastic transition
- •broad line radio galaxies
- •broad line region
- •brown dwarf
- •Brunt frequency
- •Brunt–Väisälä frequency
- •B star
- •bulkhead
- •bulk modulus
- •bulk parameters
- •buoyancy
- •buoyancy frequency
- •buoyancy Reynolds number
- •buoyancy scale
- •buoyancy scaling
- •buoyancy subrange
- •Bureau International des Poids et Mesures (BIPM)
- •Burgers vector (b)
- •burst
- •Buys Ballots Law
- •b-value
- •Byerlee’s law
- •bypass
- •cabbeling
- •cable
- •caldera
- •calendar
- •California current
- •California nebula
- •Callan–Rubakov effect
- •Callisto
- •calorie
- •Calypso
- •Canary current
- •candela
- •canonical momentum
- •canonical transformation
- •Canopus
- •cap cloud
- •CAPE (Convective Available Potential Energy)
- •Capella
- •capillarity correction
- •capillary fringe
- •capillary wave
- •carbon-14 dating
- •carbonaceous chondrite
- •carbon burning
- •carbon cycle
- •carbon dioxide
- •carbon monoxide
- •carbon star
- •Caribbean current
- •Carme
- •Carnot cycle
- •Carnot engine
- •Carrington longitude
- •Carrington rotation
- •Cartesian coordinates
- •Cartesian coordinates [in a plane]
- •Cartesian coordinates [in space]
- •case 1 water
- •case 2 water
- •Cassegrainian
- •Cassini
- •Cassini’s division
- •Cassiopeia A
- •Castor
- •Castor, John I.
- •cataclysmic variable (cataclysmic binary)
- •cataclysmic variable [binary models of]
- •cataclysmic variable [galactic distribution]
- •cataclysmic variable [outbursts]
- •cataclysmic variables [physical parameters]
- •catastrophic formation of solar system
- •Cauchy singularity
- •causal boundary
- •causal curve
- •causal future/past
- •causality relations
- •cavity, magnetic
- •cD galaxies
- •Celaeno
- •celerity
- •celerity
- •celestial equator
- •celestial poles
- •celestial sphere
- •Celsius, Anders
- •Celsius scale
- •Centaur
- •Centaurus A (Cen A)
- •center of mass
- •Centigrade scale
- •centroid moment tensor (CMT)
- •centimeter burst
- •central meridian passage
- •central peak
- •central pit
- •centrifugal force
- •Cepheid variable
- •Cerenkov radiation
- •Ceres
- •CGS (Centimeter-Gram-Second)
- •chalcophile
- •Challenger Deep
- •Chandler wobble
- •Chandrasekhar limit (or Chandrasekhar mass)
- •chaos
- •chaotic cosmology
- •chaotic system
- •chaotic terrain
- •Chapman layer
- •characteristic earthquake
- •characteristics, method of
- •charge exchange
- •Charles’ Law
- •Charon
- •chemically peculiar star
- •chenier
- •chlorinity
- •chlorophyll
- •chondrite
- •chondrite, carbonaceous
- •chondrite, ordinary
- •chondrule
- •Christoffel symbol
- •chromatic aberration
- •chromosphere
- •chromospheric evaporation
- •chromospheric heating
- •chronological future/past
- •CHUMP (Charged Hypothetical Ultra Massive Particle)
- •cigar distribution
- •circulation
- •cirrus
- •civil time
- •civil twilight
- •Clapeyron–Clausius equation
- •clapotis
- •classical radius of electron
- •clathrate
- •clay
- •clear air turbulence (CAT)
- •cleft, polar
- •climate regimes
- •climatic optimum
- •closed magnetosphere
- •closed universe
- •clump star
- •CNO cycle (or tricycle)
- •cnoidal wave
- •coastal jet
- •coastally trapped waves
- •coastal ocean
- •coastal upwelling or downwelling
- •Coastal Zone Color Scanner (CZCS)
- •cobble
- •coble creep (grain-boundary diffusion creep)
- •cobpoint
- •coda wave
- •coesite
- •cohesionless
- •cohesive sediment
- •cold dark matter
- •cold front
- •cold plasma
- •Coleman–Weinberg potential
- •collision boundary
- •collisionless shock
- •colored dissolved organic matter (CDOM)
- •color excess
- •color index
- •color-magnitude diagram
- •color temperature
- •coma
- •comet
- •comet(s): chemical composition of
- •comet(s): dirty iceball or snowball model
- •comet(s): missions to
- •comminution
- •common envelope
- •common envelope binary
- •comoving frame
- •compact group of galaxies
- •compaction
- •compact steep spectrum radio sources
- •compensation
- •compensation depth
- •composite volcanos
- •compound channel
- •compressibility
- •Compton, Arthur H.
- •Compton cooling
- •Compton scattering
- •computational relativity
- •conditional unstability
- •conducting string
- •conduction
- •conductive heat transfer
- •conductivity of water
- •conformal tensor
- •congruence
- •conical spacetime
- •conics
- •conic section
- •conjugate depths
- •conjunction
- •connection
- •connection longitude
- •Conrad discontinuity
- •conservation of angular momentum
- •conservation of energy
- •conservation of momentum
- •conservative system
- •constellation
- •constitutive law of frictional sliding
- •constraint equations
- •contact binary
- •continent
- •continental climate
- •continental collision
- •continental drift
- •continental margin
- •continental plate
- •continental shelf
- •continental shelf waves
- •continuum
- •contravariant vector
- •convection
- •convection zone
- •convective adjustment
- •convective cloud
- •convective heat transfer
- •convective instability
- •convective scaling
- •convective turbulence
- •convergent boundary
- •convergent plate boundaries
- •Coordinated Universal Time (UTC)
- •coordinate singularity
- •coordinate system
- •coordinate time
- •coordinate transformation in special relativity
- •Copernicus, Nicholas
- •coplanarity theorem
- •Cordelia
- •cordillera
- •core
- •core collapse
- •core convection
- •core-dominated quasars
- •core-mantle coupling
- •Coriolis
- •Coriolis effect (Coriolis force)
- •Coriolis, Gaspard Gustave de
- •Coriolis parameter
- •corner frequency
- •corona
- •coronagraph
- •Coronal Diagnostic Spectrometer (CDS)
- •coronal dimming
- •coronal heating
- •coronal hole
- •coronal lines
- •coronal loops
- •coronal mass ejection (CME)
- •coronal rain
- •coronal transients
- •coronal trap
- •corotating interaction region
- •correlation length
- •coseismic deformation
- •cosine collector
- •cosmic abundance
- •cosmic censorship
- •cosmic microwave background
- •cosmic microwave background, spectral distortions
- •cosmic nucleosynthesis
- •cosmic phase transition
- •cosmic rays
- •cosmic spring
- •cosmic string
- •cosmic texture
- •cosmic topological defect
- •cosmochemistry
- •cosmogenic nuclides
- •cosmological constant
- •cosmological constant problem
- •cosmological model
- •cosmological principle
- •cosmology
- •coude focus
- •coulomb
- •Coulomb collisions
- •Coulter counter
- •Courant number
- •covariant derivative
- •covariant vector
- •Cox number
- •CP problem
- •Crab Nebula
- •Crab Pulsar
- •CRAND (Cosmic Ray Albedo Neutron Decay)
- •crater
- •crater depth-diameter plots
- •cratering rates
- •cratering record
- •crater number: index of age
- •crater production function
- •crater saturation
- •craton
- •creep
- •Cressida
- •critical current (cosmic string)
- •critical density
- •critical depth
- •critical frequency
- •critical level
- •critical phenomena in gravitational collapse
- •critical point
- •critical temperature
- •critical velocity
- •cross helicity
- •cross-section
- •cross-shore
- •cross slip
- •cross waves
- •crust
- •crustal deformation
- •crustal movement
- •crystal
- •crystallization age
- •CTRS
- •cumulative size-frequency curves
- •cumulus
- •Curie (Ci)
- •Curie point
- •Curie point survey
- •curl
- •current carrier (cosmic string)
- •current, curvature
- •current generation (cosmic string)
- •current, gradient
- •current instability (cosmic string)
- •current, magnetization
- •current, polarization
- •current quenching
- •current screening (cosmic string)
- •current sheet
- •curvature
- •curvature invariant
- •curvature tensor
- •curve
- •curved space-time
- •curve of growth
- •curvilinear coordinates
- •curvilinear coordinates [in a plane]
- •curvilinear coordinates [in space]
- •cusp (cosmic string)
- •cusp, polar
- •cutoff energy
- •cyclic coordinate
- •cyclone
- •cyclongenesis
- •cyclotron damping and instability
- •cyclotron frequency
- •cyclotron radius
- •Cygnus A
- •Cygnus Loop
- •cynthion
- •Cytherean
- •Dalton’s Law
- •Darcy’s law
- •Darcy–Weisbach friction factor
- •dark cloud
- •dark matter
- •dark matter, cold
- •dark matter, hot
- •dark nebula
- •dart leader
- •data assimulation
- •Davidson current
- •daylight savings time
- •dead zone
- •Deep Space 1 (DS1)
- •Deep Space 2
- •Deep Space Network (DSN)
- •deep water wave
- •defect
- •deferent
- •deformation radius
- •degeneracy
- •degree (temperature)
- •de Hoffman–Teller frame
- •Deimos
- •delta
- •Delta Scuti stars
- •Delta surface approximation
- •density
- •density current
- •density inversion
- •depleted mantle
- •depletion layer
- •depth of compensation
- •Derrick theorem
- •Debye length
- •decelleration parameter
- •decibel
- •declination
- •decollement
- •deep(-focus) earthquake
- •Descartes ray
- •Desdemona
- •deSitter Universe
- •Despina
- •detritus
- •deuterium
- •deuterium burning
- •de Vaucouleurs’ law
- •deviative absorption
- •deviatoric strain
- •deviatoric stress
- •dew point
- •dextral fault
- •diapir
- •diapycnal
- •dichotomy of Mars
- •diel
- •dielectric strength
- •differential charging
- •differential diffusion
- •differential emission measure
- •differential heating/cooling
- •differential rotation
- •differentiation
- •diffraction
- •diffraction grating
- •diffuse aurora
- •diffuse galactic light
- •diffuse interstellar bands (DIBs)
- •diffusion
- •diffusion-convection equation
- •diffusion creep
- •diffusion, in momentum space
- •diffusion, in pitch angle space
- •diffusively stable
- •diffusive regime
- •diffusive shock acceleration
- •dike
- •dilatancy model
- •dilatation of time-Lorentz transformation
- •dilaton
- •dilaton gravity
- •dilatonic black hole
- •dilution
- •dimension
- •dimensional analysis
- •dimensional transmutation
- •Dione
- •dip-equator
- •dip, magnetic
- •dip slip fault
- •disconnection event
- •discordant redshift
- •discrete aurora
- •discrimination (seismic)
- •disk warp
- •dislocation climb
- •dislocation creep
- •dislocation energy (self-energy of dislocation)
- •dislocation glide
- •disparition brusque
- •dispersion
- •dispersionless injection
- •dispersion measure (DM)
- •dispersive
- •displacement vector
- •dissipation
- •dissipation of temperature variance
- •dissipation of turbulent kinetic energy
- •dissipation range
- •dissipation rate
- •dissolved organic matter
- •distance indicator
- •distance modulus
- •diurnal
- •diurnal motion
- •divergence
- •divergence law for irradiance
- •divergence theorem
- •divergent boundary
- •divergent plate boundary
- •diversity reception
- •divided bar
- •D-layer
- •dog days
- •doldrums
- •domain of dependence
- •domain of outer communication
- •domain wall
- •dominant energy condition
- •Doodson number
- •Doppler beaming
- •Doppler broadening
- •Doppler dimming
- •Doppler effect
- •Doppler shift
- •double couple
- •double diffusion
- •double probe
- •double star
- •downward (upward) radiance mean cosine
- •downwelling
- •draconitic month
- •dragging phenomenon
- •dredge
- •D region
- •drift
- •drift, curvature
- •drift, electric
- •drift, gradient
- •drift, magnetization
- •driftmeter
- •drift, polarization
- •driven shock
- •drizzle
- •drumlins
- •drunkards walk
- •dry-adiabatic lapse
- •dry freeze
- •duality in elastic string models
- •ductile behavior
- •dune
- •dusk
- •dust
- •dust storm
- •dust storm (Mars)
- •dwarf galaxy
- •dwarf nova
- •dwarf spheroidal galaxies
- •dynamical friction
- •dynamical time (DT)
- •dynamic height
- •dynamic meter
- •dynamic pressure
- •dynamic recrystallization
- •dynamics
- •dynamic viscosity
- •dynamo
- •Eady, E.T.
- •Eady model
- •Earth
- •earth orientation parameters (EOP)
- •earthquake
- •earthquake intensity
- •earthquake magnitude
- •earthquake moment
- •earthquake precursor
- •earthquake prediction
- •earthquake swarm
- •earth rotation parameters (ERP)
- •earthshine
- •East Australian current
- •East Greenland current
- •easy access region
- •ebb current
- •ebb delta
- •ebb shoal
- •ebb tide
- •eccentric
- •eccentric dipole
- •eccentricity
- •echelle spectrograph
- •echo sounder
- •eclipse
- •eclipse year
- •eclipsing binary
- •ecliptic
- •Eddington approximation
- •Eddington limit
- •Eddington luminosity
- •Eddington ratio
- •eddy
- •eddy correlation method
- •eddy diffusivity
- •eddy-resolving
- •eddy viscosity
- •edge wave
- •effective charge
- •effective couplings
- •effective pressure
- •effective stress
- •effective temperature
- •eigenray
- •eigenvalue
- •eikonal approximation
- •einstein
- •Einstein equations
- •Einstein summation convention
- •Einstein tensor
- •Einstein Universe
- •ejecta
- •Ekman convergence
- •Ekman layer
- •Ekman mass transport
- •Ekman pumping
- •Elara
- •elastic deformation
- •elastic limit
- •elastic lithosphere
- •elastic modulus
- •elastic rebound
- •elastic string model
- •E-layer screening
- •Electra
- •electric drift
- •electric regime (cosmic string)
- •electroglow
- •electromagnetic current meter
- •electromagnetic induction
- •electromagnetic radiation
- •electromagnetism
- •electron
- •electron precipitation
- •electron temperature
- •electron volt
- •electrostatic unit
- •electrovacuum
- •element
- •elemental abundances: general
- •elemental abundances: in minerals
- •elemental abundances: in organics
- •elemental abundances: of comet(s)
- •elevation
- •elliptical galaxies
- •Ellison scale
- •elongation
- •elongation
- •elves
- •Elysium Province
- •embedded defect
- •emission line
- •emission lines: interstellar and cometary
- •emissivity
- •empirical model of the magnetosphere
- •Enceladus
- •endothermic
- •energetic particles
- •energetic particles in interplanetary space
- •Energetic Particle Population in the Heliosphere
- •energetic storm particles
- •energy
- •energy-containing scale
- •energy grade line
- •energy per unit length (cosmic string)
- •enthalpy
- •entrainment
- •entropy
- •environmental lapse rate
- •eolian
- •Eötvös experiment
- •epeiric sea
- •ephemeris
- •ephemeris time (ET)
- •epicenter
- •epicentral distance
- •epicycle
- •Epimetheus
- •epoch
- •equant
- •equation of continuity
- •equation of state
- •equation of state (cosmic string)
- •equation of state of pure water
- •equation of time
- •equator
- •equator, geomagnetic
- •equatorial anomaly
- •equatorial bulge
- •equatorial cold tongue
- •equatorial convergence zone
- •equatorial easterlies
- •equatorial electrojet
- •equatorial Kelvin wave
- •equatorial surface of the magnetosphere
- •equatorial undercurrent
- •equatorial upwelling
- •equatorial waveguide
- •equatorial waves
- •equilibrium
- •equilibrium range
- •equilibrium space-times
- •equilibrium tide (gravitational tide)
- •equilibrium vapor pressure
- •equinox
- •equivalence principle
- •Eratosthenes
- •E region
- •ergodic motion
- •ergoregion
- •ergs
- •Ernst equation (1967)
- •erosion
- •Ertel potential vorticity
- •eruption
- •eruptive prominence
- •escape velocity
- •eskers
- •estuary
- •eternal black hole
- •Eudoxos of Cnidus
- •Euler equations
- •Eulerian
- •Eulerian coordinates
- •Eulerian representation
- •Eulerian velocity
- •Euler, Leonhard
- •Euler pole
- •Euler potentials
- •euphotic depth
- •euphotic zone
- •euphotic zone midpoint
- •Europa
- •eutrophic water
- •evaporation (
- •evapotranspiration (
- •evening cloud (Mars)
- •event horizon
- •Evershed effect
- •evolutionary track
- •exact solution
- •excitation temperature
- •exosphere
- •exotic terrane
- •expansion
- •expansion of the universe
- •experimental craters
- •extended object
- •extensions of space-times
- •extinction and reddening
- •extratropical storm
- •extreme ultraviolet (EUV)
- •extreme ultraviolet imaging telescope (EIT)
- •eye (of a storm)
- •F1-layer
- •F2-layer
- •facula
- •fading
- •failed arm
- •faint young sun paradox
- •Falkland current
- •fallout
- •fall speed (velocity)
- •Fanaroff–Riley (FR) class I and II radio galaxies
- •Faraday Cup
- •Faraday effect
- •Faraday rotation
- •fast magnetohydrodynamic shock
- •fast shock wave
- •fast solar wind
- •Fata Morgana
- •fathom
- •fault
- •fault constitutive law
- •fault gouge
- •fault parameter
- •fault plane solution
- •fault scarp
- •F corona
- •feeder beach
- •feldspar
- •Fermat’s principle
- •fermion
- •fermionic zero mode
- •Ferrel cell
- •fertile mantle
- •fetch
- •FG Sagittae star
- •Fick’s second law
- •field line motion
- •finestructure
- •fjord
- •FK Comae stars
- •flocculation
- •Flora
- •Florida current
- •fluxrope
- •focal length
- •focal mechanisms
- •focus
- •focused transport equation
- •focusing
- •foehn
- •folds
- •following spot
- •Fomalhaut
- •forbidden lines
- •forbidden orbits
- •forbidden region
- •Forbush decrease
- •force
- •force balance
- •Forchhammer’s Principle
- •foreshore
- •foreshock
- •forecasting (wave)
- •forearc sliver
- •forearc basin
- •Foucault pendulum
- •four-velocity
- •fractal
- •fractionation
- •fracture
- •fracture zone
- •fragmentation
- •frame dragging
- •Fraunhofer lines
- •free-air gravity anomaly
- •free atmosphere
- •free bodies
- •free-bound continuum emission
- •free convection
- •free-free continuum emission
- •free oscillations
- •freeze
- •F region
- •Fresnel zone
- •fretted channels
- •fretted terrain
- •friction
- •friction factor
- •friction slope
- •friction velocity
- •Friedmann, Aleksandr Aleksandrovich
- •Friedmann–Lemaître cosmological models
- •fringe (interference)
- •front
- •frontogenesis
- •frost point
- •Froude number
- •F star
- •Fukushima’s theorem
- •fully arisen sea
- •fulvic substance
- •fundamental tensors of a worldsheet
- •future/past causal horizon
- •future/past event horizon
- •gabbro
- •galactic bulge
- •galactic cluster
- •galactic coordinates
- •galactic disk
- •galactic globular cluster
- •galactic noise
- •galactic wind
- •Galatea
- •galaxy
- •Galilean invariance
- •Galilean relativity
- •Galilean transformation
- •Galilei, Galileo
- •Galileo spacecraft
- •Galiliean satellite
- •gallium
- •gamma
- •gamma ray burst (GRB)
- •gamma-ray burst, black hole accretion disks
- •gamma-ray burst, classical
- •gamma-ray burst, cosmological mechanisms
- •gamma-ray burst, galactic mechanisms
- •gamma-ray burst, GRB 970508
- •gamma-ray burst, hypernova
- •gamma-ray burst, mechanisms
- •gamma-ray burst models, collapsar
- •gamma-ray burst models, helium merger
- •gamma-ray burst, soft gamma-ray repeaters
- •Ganymede
- •garden hose angle
- •gas constant
- •gaseous shocks
- •gas thermometer
- •gauge
- •gauge pressure
- •Gauss
- •gegenschein
- •gelbstoff
- •general circulation model (GCM)
- •general relativity
- •geocentric
- •geocentric coordinate time
- •geocentric latitude
- •geocorona
- •geodesic
- •geodesic completeness
- •geodesy
- •geodetic latitude
- •geodynamics
- •geodynamo
- •Geographus
- •geoid
- •geoid anomalies
- •geomagnetic activity
- •geomagnetic dip equator
- •geomagnetic disturbance
- •geomagnetic elements
- •geomagnetic indices
- •geomagnetic jerk
- •geomagnetic potential
- •geomagnetic storm
- •geometrodynamics
- •geophysics
- •geopotential
- •geopotential surface
- •geospace
- •geostrophic adjustment
- •geostrophic approximation
- •geostrophic balance
- •geostrophy
- •Geosynchronous Operational Environmental Satellites (GOES)
- •geosynchronous orbit
- •geotherm
- •geothermal energy
- •geothermal gradient
- •Geroch group
- •Gershun’s law
- •Gershun tube
- •giant branch
- •giant cells
- •giant planet
- •Gibbs free energy (Gibbs Potential)
- •gilvin
- •Ginzburg temperature
- •glacial
- •glaciation
- •glacier
- •global loop oscillation
- •globally hyperbolic space-time
- •global positioning system (GPS)
- •global seismology
- •global thermohaline circulation
- •global topological defect
- •globular cluster
- •gnomon
- •Goddard, Robert H.
- •Goldstone boson
- •Goldstone model
- •Goldstone theorem
- •Gondwanaland
- •graben
- •GRACE (Gravity Recovery and Climate Experiment)
- •gradient
- •gradient drift
- •gradient Richardson number
- •gradual commencement storm
- •grain-boundary migration
- •grain-boundary sliding
- •grain chemistry: dense interstellar
- •grain chemistry: diffuse interstellar
- •grains: in other galaxies
- •grains, interstellar: destruction and formation
- •grains, interstellar: size and composition
- •grain size
- •grain size analysis
- •grand potential
- •granulation
- •grating spectrograph
- •gravitation
- •gravitational collapse
- •gravitational constant G
- •gravitational equations
- •gravitational instability
- •gravitational lens
- •gravitational multipole moments
- •gravitational perturbations
- •gravitational potential
- •gravitational radius
- •gravitational redshift
- •gravitational wave
- •graviton
- •gravity
- •gravity anomaly
- •gravity assist
- •grazing incidence optics
- •Great Attractor
- •Great Red Spot
- •great salinity anomaly (GSA)
- •Greenhouse Effect
- •greenhouse gases
- •green line
- •Green’s theorem
- •Greenwich mean (solar) time (GMT)
- •Greenwich sidereal date (GSD)
- •Gregorian
- •Gregorian calendar
- •grism
- •groin
- •gross photosynthetic rate
- •ground level event (GLE)
- •ground stroke
- •groundwater
- •group velocity
- •growing season
- •G star
- •Guiana current
- •guiding center
- •guiding center approximation (GCA)
- •gulf stream
- •guyot
- •gyrofrequency
- •gyroradius
- •Hadley (cell) circulation
- •Hadley cell
- •hadron
- •hail
- •hailstone
- •Hale cycle
- •Hale–Nicholson Polarity Law
- •Halley’s comet
- •halo
- •halocline
- •Hamiltonian
- •Hamilton–Jacobi Theory
- •hard freeze
- •hard radiation
- •Hard X-ray Telescope (HXT)
- •harmonic analysis
- •harmonic model
- •heat capacity
- •Heaviside, Oliver (1850–1925)
- •heavy minerals
- •Hebe
- •Helene
- •heliacal rising
- •helicity
- •heliocentric
- •heliopause
- •helioseismology
- •heliosheath
- •Helios mission
- •heliosphere
- •heliospheric current sheet (HCS)
- •heliospheric stream structure
- •heliospheric termination shock
- •helium
- •helium burning
- •helmet streamer
- •Helmholtz free energy (free energy)
- •Helmholtz theorem
- •Herbig Ae/Be star
- •herringbone burst
- •Hertz (Hz)
- •Hertzsprung–Russell diagram
- •Hesperian
- •Higgs mechanism
- •higher derivative theories
- •highlands, lunar
- •high-pressure and high-temperature experiment
- •HI region
- •Himalia
- •hindcasting (wave)
- •historical climate
- •hoarfrost
- •Holmberg radius
- •homogeneity
- •homologous temperature (
- •homothety
- •homotopy group
- •hopper dredge
- •horizon
- •horizontal branch star
- •horse latitudes
- •horst
- •hot dark matter
- •hot spot
- •hot towers
- •hour angle
- •Hubble diagram
- •Hubble parameter (Hubble constant)
- •Hubble radius
- •Hubble–Reynolds law
- •Hubble sequence
- •Hubble’s law
- •Hubble space telescope (HST)
- •Hubble time
- •humic substance
- •hurricane
- •Huygens principle
- •Hyades
- •hybrid topological defect
- •hydraulic head (piezometric head)
- •hydraulic conductivity (
- •hydraulic depth
- •hydraulic-fracturing method
- •hydraulic gradient (
- •hydraulic head (
- •hydraulic jump
- •hydraulic radius
- •hydraulic routing
- •hydraulic transmissivity
- •hydrodynamic
- •hydrodynamic instability
- •hydrogen
- •hydrogen burning
- •hydrograph
- •hydrographic survey
- •hydrologic equation
- •hydromagnetic
- •hydromagnetics
- •hydromagnetic shock wave
- •hydromagnetic turbulence
- •hydromagnetic wave
- •hydrosphere
- •hydrostatic
- •hydrostatic approximation
- •hydrostatic equation
- •hydrostatic equilibrium
- •hydrostatic pressure
- •Hygiea
- •hygrometer
- •Hyperion
- •hypersonic
- •hysteresis
- •Iapetus
- •Icarus
- •ideal gas
- •ideal gas [equation of state]
- •igneous
- •igneous rocks
- •illuminance (lux)
- •immersed weight
- •impact basin
- •impact crater
- •impact crater ejecta
- •impact creep
- •impact excavation phase
- •impact polarization
- •impact velocities
- •impermeable
- •inclination
- •incompatible element
- •index, geomagnetic activity
- •index of refraction (n)
- •indices, geomagnetic
- •induced Compton scattering
- •induced gravity
- •inelastic collision
- •inelastic scattering of radiation
- •inertia
- •inertial-convective subrange
- •inertial coordinate system
- •inertial frequency
- •inertial instability
- •inertial mass
- •inertial oscillation
- •inertial subrange
- •inferior conjunction
- •inferior mirage
- •infrared
- •infrasound
- •inherent optical property (IOP)
- •inhomogeneous models (of the universe)
- •initial condition
- •initial data
- •initial mass function (IMF)
- •injection boundary
- •inner core
- •inner radiation belt
- •inshore
- •insolation
- •intensity
- •interaction of galaxies
- •intercloud medium
- •intercommutation
- •interface-controlled creep
- •interference fading
- •interglacial
- •interior Schwarzschild space-time
- •intermediate shock
- •intermittency
- •internal energy
- •internal friction angle
- •internal wave
- •International Atomic Time (TAI)
- •international dateline
- •International System of Units (SI)
- •International Temperature Scale (ITS-90)
- •interplanetary dust particles (IDPs)
- •interplanetary magnetic sector
- •interplanetary propagation
- •interplanetary scintillation (IPS)
- •interplanetary scintillation (IPS) observations
- •interplanetary sector structure
- •interplanetary shock wave
- •interplanetary stream structure
- •interplate earthquake
- •interstellar clouds
- •interstellar dust
- •interstellar gas
- •interstellar medium
- •interstellar molecules
- •intertropical convergence zone (ITCZ)
- •intraplate earthquake
- •intrinsic permeability (
- •intrusion
- •invariant
- •inverse Compton effect
- •inverse Compton radiation
- •inverse problems
- •inverse theory
- •inverted barometer effect (inverse barometer response)
- •ion acoustic waves
- •ionization
- •ionization equilibrium
- •ionizing radiation
- •ionogram
- •ionosonde
- •ionosphere
- •ionospheric absorption
- •ionospheric index
- •ionospheric polarization
- •ionospheric propagation mode
- •ionospheric radio propagation path
- •ionospheric refraction
- •ionospheric regions
- •ionospheric sounder
- •ionospheric sounding
- •ionospheric storm
- •ionospheric variability
- •ion torus
- •ion trap
- •IRAF (Image Reduction and Analysis Facility)
- •Iris
- •IRIS (Incorporated Research Institutions for Seismology)
- •iron
- •line
- •iron meteorite
- •irradiance
- •irregular galaxies
- •irregular waves
- •irribarren number
- •irrotational
- •ISC (International Seismological Centre)
- •isentropic coordinate
- •island arc
- •isobar
- •isobaric coordinate
- •isogonic lines
- •isoline
- •isometry
- •isopycnal processes
- •isostacy
- •isotherm
- •isothermal atmosphere
- •isotope
- •isotope delta value (
- •isotope fractionation factor (
- •isotropic
- •isotropic turbulence
- •isotropy
- •Jacobian
- •Jacobi ellipsoid
- •Jansky (Jy)
- •Janus
- •Japan current
- •Jeans escape
- •Jeans instability
- •Jeans length
- •Jerlov water type
- •jet stream
- •jetty
- •joints
- •JONSWAP
- •Joule (J)
- •Joule heating
- •Jovian planet
- •Julian calendar
- •Julian Date Systems Prior to 1972
- •Transformations among JD, MJD, and Gregorian calendar date
- •Julian year
- •Juliet
- •Junge particle distribution
- •Juno
- •Jupiter
- •katabatic wind
- •k-correction
- •Kelvin–Helmholtz instability
- •Kelvin material
- •Kelvin temperature scale
- •Kelvin (Thompson) circulation theorem
- •Keplerian map
- •Kepler shear
- •Kepler’s laws
- •Kepler’s supernova (SN1604, 3C358)
- •Kerr black hole
- •Kerr metric
- •Keulegan–Carpenter Number
- •K-function
- •Kibble mechanism
- •Kibel, I.A.
- •Killing horizon
- •Killing tensor
- •Killing vector
- •K index
- •kinematical invariants
- •kinematics
- •kinematic viscosity
- •kinetic energy
- •kinetic temperature
- •kink
- •Kirchoff’s law
- •Kirkwood gaps
- •knoll
- •knot
- •Knudsen number
- •Kolmogorov scale (Kolmogorov microscale)
- •Kolmogorov spectrum
- •Kp index
- •KREEP
- •Kruskal extension (1960)
- •krypton (Kr)
- •K star
- •Kuiper belt
- •Kuiper belt object, trans-Neptunian object, Edgeworth–Kuiper object
- •Kuroshio
- •lagoon
- •Lagrange points
- •Lagrangian
- •Lagrangian coordinates
- •Lagrangian coordinates
- •Lagrangian representation
- •Lagrangian velocity
- •Laing–Garrington effect
- •Lambertian surface
- •Lambert’s law
- •Lamé constants
- •Landau damping and instability
- •Langmuir circulation
- •Langmuir waves
- •Laplace equation
- •lapse rate
- •Large Magellanic Cloud (LMC)
- •large-scale
- •Larissa
- •Larmor frequency
- •last scattering
- •latent heat
- •latitude
- •lattice preferred orientation (LPO)
- •Laurasia
- •lava
- •law of equal areas
- •law-of-the-wall layer
- •law-of-the-wall scaling
- •laws of black hole physics
- •layering
- •leader spot
- •leap second
- •leap year
- •Leda
- •lee wave
- •left-lateral strike-slip fault
- •Lemaître, Georges
- •Law-of-the-Wall Scaling Relations
- •Lemaître–Tolman cosmological model
- •length of day
- •lens
- •Lense–Thirring precession
- •lepton
- •leveling (survey)
- •Lewis number
- •Lg wave
- •libration
- •Lie derivative
- •lifting condensation level
- •light bridge
- •light cone
- •light-curve
- •light-harvesting complex
- •lightlike current (cosmic string)
- •lightning
- •light pollution
- •light year (l.y.)
- •LIGO (Laser Interferometric Gravitational Observatory)
- •limb
- •limb brightening
- •limb darkening
- •Lindblad resonance
- •linearized gravitation
- •linear momentum
- •linear wave theory
- •line of apsides
- •line of nodes
- •LINER
- •line squall
- •line wing
- •Liouville’s theorem (the equation of continuity)
- •liquefaction
- •LISA (Laser Interferometric Space Antenna)
- •lithophile
- •lithosphere
- •lithostatic pressure
- •little ice age
- •littoral
- •littoral barrier
- •littoral cell
- •littoral current
- •littoral drift
- •littoral drift rose
- •littoral transport
- •lobe
- •lobe dominated quasars
- •lobes, high latitude
- •local acceleration of gravity
- •Local Group
- •local thermodynamic equilibrium (LTE)
- •local topological defect
- •loess
- •Long Duration Event (LDE)
- •longitude, terrestrial
- •longitudinal invariant
- •longitudinal wave
- •long period variables
- •longshore bar
- •longshore current
- •longshore sediment transport
- •long slit spectroscopy
- •Lorentz boost
- •Lorentz factor,
- •Lorentz–Fitzgerald contraction
- •Lorentz force equation
- •Lorentzian metric
- •Lorentz invariance
- •Lorentz transformation
- •Lorentz transformation [implications]
- •Love numbers
- •Love wave
- •lower hybrid waves
- •lower mantle
- •Lowes power spectrum
- •Lowest Useable Frequency (LUF)
- •Low frequency radio emission from planets
- •luminosity class
- •luminosity distance
- •luminosity function of galaxies
- •luminous blue variables
- •Luminous power (lumens)
- •lunar eclipse
- •lunar highlands
- •lunar libration
- •Lunar Maria
- •lunar mascons
- •lunar meteorites
- •Lunar Prospector
- •Lunar Rille
- •Lyapunov exponent
- •Lyman limit
- •Lyman series
- •Lysithea
- •Mach, Ernst (1838–1916)
- •Mach’s principle
- •Maclaurin Spheroid
- •macroscopic description (cosmic string)
- •Madden–Julian oscillation (MJO)
- •Magellanic Clouds
- •Magellan Mission
- •magma
- •magma chamber
- •magmatic water
- •magnetic anomaly
- •magnetic bay
- •magnetic carpet
- •magnetic cloud
- •magnetic crochet
- •magnetic declination
- •magnetic diffusivity
- •magnetic dipole
- •magnetic helicity
- •magnetic inclination
- •magnetic latitude
- •magnetic local time (MLT)
- •magnetic longitude
- •magnetic mirror
- •magnetic pole
- •magnetic pressure
- •magnetic reconnection
- •magnetic regime (cosmic string)
- •magnetic reversal
- •magnetic Reynolds number
- •magnetic secular variation
- •magnetic shear
- •magnetic tension
- •magnetism
- •magnetoacoustic wave
- •magnetogram
- •magnetohydrodynamics (MHD)
- •magnetometer
- •magnetopause
- •magnetosonic wave
- •magnetosphere
- •magnetospheric substorms
- •magnetostratigraphy
- •magnetostrophic
- •magnetotail
- •magnitude
- •Maia
- •main sequence star
- •major axis
- •Manning’s equation
- •manometer
- •mantle
- •mantle convection
- •mantle transition zone
- •March 5th event
- •mare
- •Marianas Trench
- •marine snow
- •Markarian galaxies
- •Mars
- •Mars Climate Orbiter (MCO)
- •Mars Global Surveyor (MGS)
- •Mars Microphone
- •Mars Microprobe
- •Mars Polar Lander (MPL)
- •Martian geophysical epochs
- •Martian meteorites
- •mascon
- •maser
- •mass-defect
- •mass extinctions
- •massive ghost
- •mass-luminosity relation
- •mass transfer
- •mass wasting
- •matter density perturbations
- •Maunder Minimum
- •maximal extension of a space-time
- •maximally rotating black hole
- •maximally symmetric space
- •Maximum Observable Frequency (MOF)
- •Maxwell–Boltzmann distribution
- •Maxwell material
- •mean anomaly, M
- •mean celestial equator
- •mean celestial pole
- •mean cosine of scattering angle
- •mean diameter, mean grain size
- •mean free path
- •mean motion
- •mean motion (short-period) resonances
- •mean sea level
- •mean solar day
- •mean solar time
- •mean water level
- •mechanics
- •mechanics, Newtonian
- •median diameter, median grain size
- •Meiyu front
- •membrane paradigm
- •Mercalli intensity scale
- •Mercury
- •merger
- •merging, magnetic
- •Merian’s equation
- •meridian
- •meridional
- •meromixis
- •Merope
- •mesogranulation
- •meson
- •mesoscale
- •mesosphere
- •mesotrophic water
- •Messier
- •metallic hydrogen
- •metallicity
- •metamorphism
- •meteor
- •meteorite
- •meteorite parent body
- •meteoroid
- •meteor shower
- •methane
- •Metis
- •metric
- •metricity of covariant derivative
- •metric radio burst
- •MHD condition
- •MHD simulation
- •Michelson Doppler Imager/Solar Oscillations Investigation (MDI/SOI)
- •Michelson–Morley experiment
- •Miche–Rundgren theory
- •micrometeorite
- •microstructure
- •microwave background radiation
- •microwave burst
- •Mie scattering
- •Mie size parameter
- •migration (seismic)
- •Milankovich cycle
- •Miles–Phillips–Hasselmann theory
- •millibar
- •Mimas
- •minimal coupling
- •minisuperspace
- •minor axis
- •Mira
- •mirage
- •Miranda
- •mirror instability
- •mixed layer
- •mixing length
- •mixing ratio
- •Mixmaster universe
- •M magnitude
- •model atmosphere
- •mode water
- •modulation of galactic cosmic rays
- •Mohr’s circle
- •moist lapse rate
- •molar gas constant (
- •molecular cloud
- •molecular torus
- •moment
- •moment of inertia
- •Monin–Obukhov length
- •monopole
- •monopole annihilation
- •monopole excess problem
- •monsoon
- •monsoon climate
- •month
- •moody diagram
- •moon
- •moonbow
- •moraines
- •Moreton wave
- •morning cloud (Mars)
- •morphodynamics
- •morphology-density relationship
- •mountain climate
- •moving magnetic features
- •M star
- •MUF fading
- •multicell storm
- •multipath fading
- •multiple ring basins
- •multiple shock
- •mushy zone
- •nadir
- •nadir angle
- •Naiad
- •naked singularity
- •nakhlite
- •nanotesla (nT)
- •narrow emission line galaxies
- •Narrow Line Region (NLR)
- •Nasmyth universal spectrum
- •natural line broadening
- •natural remanent magnetism
- •nautical mile
- •nautical twilight
- •neap tide
- •NEAR
- •nearly diurnal free wobble
- •nebular hypothesis
- •nebular lines
- •neon
- •neon burning
- •Neptune
- •Nereid
- •net photosynthetic rate
- •network
- •Neupert effect
- •neutral equilibrium
- •neutral point
- •neutral stability
- •neutrino
- •neutrino annihilation
- •neutrino viscosity
- •neutron albedo
- •neutron star
- •neutron star kicks
- •Newman–Penrose formalism
- •New Millennium Program (NMP)
- •Newtonian
- •Newtonian cosmology
- •Newtonian gravitational constant
- •Newtonian gravity
- •Newtonian invariance
- •Newtonian relativity
- •Newtonian simultaneity
- •Newton (N)
- •Newton’s laws of motion 1.
- •Nielsen–Olesen vortex
- •nimbus
- •nitrogen
- •Noachian
- •nocturnal
- •nodal month
- •node
- •nonderivative absorption
- •nonminimal coupling
- •nonsimultaneous Big Bang
- •non-thermal line broadening
- •non-thermal radiation
- •non-thermal spectral energy distribution
- •non-topological soliton
- •nonuniqueness
- •norite
- •normal fault
- •normal incidence frame
- •normal incidence optics
- •normal modes
- •North Atlantic current
- •North Atlantic Oscillation (NAO)
- •North Equatorial current
- •Northern Plains (Mars)
- •nova
- •nuclear fusion
- •nuclear reactions
- •nuclear time scale
- •nuclear winter
- •nucleation
- •nucleus
- •nucleus (of a comet)
- •null vector
- •numerical cosmology (computational cosmology)
- •numerical model
- •numerical relativity
- •Nusselt number
- •nutation
- •obduction
- •Oberon
- •objective grating
- •objective prism
- •oblateness
- •oblique ionogram
- •oblique-slip fault
- •obliquity
- •obliquity factor
- •Occam’s razor
- •occluded front
- •occultation
- •ocean
- •ocean color
- •ocean color sensor
- •oceanic optics
- •Ockham’s razor
- •offshore
- •Olbers paradox
- •oligotrophic water
- •Olympus Mons
- •omega bands
- •One-form, 1-form
- •Oort cloud
- •open cluster
- •open magnetosphere
- •open ocean
- •open universe
- •operational MUF
- •Ophelia
- •ophiolites
- •opposition
- •optical closure in measurement
- •optical closure in models
- •optical closure in scale
- •optical constants
- •optical density
- •optical depth
- •optical distance
- •optical double star
- •optical oceanography
- •Optimum Working Frequency (OWF)
- •orbital elements
- •orogeny
- •orographic cloud
- •Orowan’s equation
- •orthogonal
- •Osborn–Cox method
- •Osborn model
- •oscillating universe
- •O star
- •outer core
- •outfall
- •outgoing longwave radiation (OLR)
- •oval equation
- •overtopping
- •overturning scale
- •overwash
- •OVV quasar
- •oxygen
- •Ozmidov scale
- •ozone
- •ozone layer
- •ozonosphere
- •package effect
- •paleoclimate
- •palimpsest
- •Pallas
- •pancake distribution
- •pancake dome
- •Pandora
- •Pangaea
- •Papapetrou spacetimes (1953)
- •paraboloid
- •parallax
- •Parker limit
- •parsec
- •partial derivative
- •partial pressure
- •particle acceleration
- •particle horizon
- •pascal (Pa)
- •Paschen series
- •Pasiphae
- •passive continental margin
- •passive earth force
- •patchiness and intermittence
- •patera
- •pathline
- •Peclet number
- •peculiar motion
- •peculiar velocity
- •peeling property
- •penetrative convection
- •Penman equation (or combination equation)
- •Penrose diagram
- •Penrose process
- •penumbra
- •percolation
- •perfect gas
- •periapsis
- •periastron
- •perihelion
- •perihelion shift
- •period
- •period-luminosity relation
- •permafrost
- •permeability
- •persistent current
- •perturbation theory
- •perturbative solution
- •Peru current
- •Petchek reconnection
- •Petrov types
- •Petzold data
- •Pfund series
- •phase
- •phase angle
- •phase frequency threshold
- •phase function
- •phase space
- •phase speed
- •phase transition
- •phase velocity
- •pheophytin
- •phi unit
- •Phobos
- •Phoebe
- •photodissociation region
- •photoelectrons
- •photoinhibition
- •photoionization
- •photometric binary
- •photometry
- •photon
- •photosphere
- •photosynthesis
- •photosynthetically available radiation
- •photosynthetic capacity
- •photosynthetic pigment
- •phytoplankton
- •piezometer
- •piezometric head
- •Pileus cloud
- •pitch angle
- •pitch angle diffusion
- •Pitot tube
- •plage
- •plagioclase (triclinic feldspar)
- •Planck constant
- •Planck length
- •Planck mass
- •Planck time
- •planet
- •planetary boundary layer
- •planetary circulation
- •planetary magnetosphere
- •planetary nebula
- •planetary radio astronomy
- •planetary rotation periods
- •planetesimal
- •plankton
- •plankton bloom
- •plasma
- •plasma frequency
- •plasma mantle
- •plasma sheet boundary layer (PSBL)
- •plasmasphere
- •plasma stress tensor
- •plastic anisotropy
- •plastic (permanent) deformation
- •plateau material
- •plate tectonics
- •platonic year
- •Pleiades
- •Pleione
- •Pluto
- •pluton
- •plutonic
- •pocket beach
- •point object
- •point spread function (PSF)
- •polar cap
- •polar cap absorption (PCA)
- •polar cap arc (or sunward arc)
- •polar cap (Mars)
- •polar cap precipitation
- •polar crown
- •polar cusp
- •polar dunes
- •polar glow
- •polar hood (Mars)
- •Polaris
- •polarization
- •polarization brightness
- •polarization fading
- •polarization state
- •polar motion
- •polar plume
- •polar wander
- •polar wind
- •pole-on magnetosphere
- •poles of Mars
- •Pole star
- •Pollux
- •poloidal/toroidal decomposition
- •polycyclic aromatic hydrocarbons (PAHs)
- •polynya
- •polytropic process
- •poorly graded sediment
- •Population I
- •Population II
- •poroelastic medium
- •Portia
- •position vector
- •positron
- •POSS
- •postglacial rebound
- •post-seismic relaxation
- •potassium-argon age
- •potential
- •potential density
- •potential energy
- •potential height
- •potential instability
- •potential temperature
- •potential theory
- •potential vorticity
- •potentiometric surface (or piezometric surface)
- •power
- •p process
- •prairie
- •Prandtl number
- •Pratt compensation
- •Pratt isostasy
- •precession
- •precession of the equinoxes
- •precipitation
- •precursor
- •pressure
- •pressure altitude
- •pressure anisotropy
- •pressure coordinate
- •pressure head (
- •primary production
- •primary productivity
- •primary rainbow
- •prime focus
- •primordial black holes
- •principal null directions
- •principal spinors
- •principle of equivalence
- •probable maximum hurricane
- •progressive vector diagram
- •progressive wave
- •Prometheus
- •prominence
- •proper time
- •proplyd
- •Proteus
- •proton
- •protostar
- •protosun
- •protuberance
- •Proxima Centauri
- •psychrometric constant
- •psychrometer
- •pseudovector
- •pseudobreakup
- •Puck
- •pulsar
- •pulsar velocities
- •pycnocline
- •pycnostad
- •pyconuclear reaction
- •pyroclastic
- •pyroxene
- •Q factor
- •quadrupole formula
- •quanta meter
- •quantization of redshift
- •quantum gravity
- •quantum yield
- •quark
- •quasar
- •quasar-galaxy association
- •quasi-biennial oscillation
- •quasi-hydrostatic approximation
- •quasi-neutral equilibrium
- •quasi-separatrix layer
- •quasi-single-scattering approximation
- •quasi stellar object
- •quasi-viscous force
- •quay
- •quenching
- •quicksand
- •quiet sun
- •radar
- •radian
- •radiance
- •radiation
- •radiation belts
- •radiation cooling
- •radiation gauge
- •radiation pressure
- •radiation tide
- •radiation zone
- •radiative-convective equilibrium
- •radiative transfer equation
- •radio absorption
- •radioactive
- •radioactive decay
- •radio burst
- •radio core
- •radio emission: types I-IV
- •Radio Frequency Bands
- •radio frequency interference (RFI)
- •radio frequency spectrum
- •radio galaxy
- •radioisotopes
- •radio lobe
- •radiometer
- •radiometric dating
- •radiometry
- •radio stars
- •radius of deformation
- •radon
- •rain
- •rainbow
- •Raman scattering
- •random waves
- •Rankine–Hugoniot relations
- •rare earth elements
- •Raychaudhuri equation
- •Rayleigh distribution
- •Rayleigh–Jeans approximation
- •Rayleigh number
- •Rayleigh scattering
- •Rayleigh wave
- •ray parameter
- •ray tracing
- •ray tracing: wave packet approximation
- •R Coronae Borealis stars
- •real singularity
- •recombination (in atomic and molecular physics)
- •recombination line
- •reconnection
- •recurrent novae
- •red giant
- •red line
- •redshift
- •Red Spot
- •reduced gravity
- •reduced gravity model
- •reef
- •reference frame
- •refracting telescope
- •refraction
- •refractive index
- •refractory
- •region of anomalous seismic intensity
- •Regolith
- •Regulus
- •relative depth
- •relative humidity
- •relativistic jets
- •relativistic time delay
- •relativity
- •relaxation time
- •residual circulation
- •resolution
- •resonance
- •resonance scattering
- •resonant absorption
- •resonant damping and instability
- •resonant layer
- •rest mass
- •retrograde motion
- •retrograde orbit
- •retrograde rotation
- •return current
- •return stroke
- •reverse fault
- •reversible process
- •revetment
- •Reynolds decomposition
- •Reynolds number (
- •Reynolds stress
- •Rhea
- •rheology
- •Ricci tensor
- •Richard’s equation
- •Richardson, Lewis F.
- •Richardson number
- •Richter magnitude scale
- •ridge push
- •ridge slide (ridge push)
- •Riemann-Christoffel tensor
- •Riemann tensor
- •Rigel
- •right ascension
- •right-hand coordinate system
- •right-lateral strike-slip fault
- •rigidity
- •rigid lid approximation
- •rille
- •Rindler observer
- •ring
- •ring current
- •ring galaxy
- •riometer
- •rip current
- •riprap
- •Robertson–Walker cosmological models
- •Robinson–Trautman space-time (1962)
- •Roche limit
- •Roche lobe
- •Rocket effect (string loop)
- •Rosalind
- •Rossby number
- •Rossby radius
- •Rossby radius of deformation
- •Rossby wave
- •rotation
- •rotational discontinuity
- •rotation curve
- •rotation of the universe
- •r process
- •RS Canum Venaticorum stars
- •runoff channels
- •runup
- •RV Tauri stars
- •Sagittarius A*
- •salinity
- •salinity inversion
- •saltation
- •salt wedge
- •sand budget
- •sand bypassing
- •sand spit
- •sand trap
- •Sargasso Sea
- •saros
- •satellite altimetry
- •Satellite Laser Ranging (SLR)
- •saturation
- •saturation adiabat
- •saturation adiabatic lapse rate
- •saturation vapor pressure
- •Saturn
- •scalar
- •scale factor
- •scaling solution (cosmic string and cosmic texture)
- •scarps
- •scatterance
- •scattered disk, scattered disk object
- •scatter-free shock acceleration
- •scattering albedo
- •scattering angle
- •scattering cross-section
- •Schmidt camera
- •Schwarzschild black hole
- •Schwarzschild metric (Schwarzschild solution)
- •Schwarzschild solution
- •Schwarzschild spacetime
- •scintillation
- •scour
- •sea level
- •seamount
- •seasonal thermocline
- •sea stack
- •seawall
- •SeaWiFS
- •Secchi depth
- •Secchi disk
- •second
- •secondary craters
- •secondary rainbow
- •second fundamental form
- •sector boundary
- •sector structure
- •secular (long-period) resonances
- •sedimentary
- •sedimentary basin
- •sediment budget
- •sediment load
- •sediment sink
- •sediment source
- •sediment transport
- •seeing
- •seiche
- •seismic coupling factor
- •seismic gap
- •seismic intensity
- •seismicity
- •seismic moment
- •seismic parameter
- •seismic wave
- •seismogram
- •seismograph
- •seismology
- •seismometer
- •seismotectonics
- •selective heating
- •self-generated turbulence
- •semiclassical gravity
- •semimajor axis
- •separator
- •separatrix
- •sequence stratigraphy
- •Sérsic–Pastoriza (1965) galaxies
- •seston
- •setdown
- •settling speed (velocity)
- •settling tube
- •setup
- •Seyfert-1 galaxies
- •Seyfert galaxies
- •sferic
- •shallow water
- •shallow water wave
- •shatter cone
- •shear
- •shear modulus
- •shear probe
- •shear stress
- •shear velocity
- •shear wave
- •shear wave splitting
- •sheet pile
- •shelf wave
- •shepherding moon
- •shepherd satellite
- •shergottite
- •shields diagram
- •shield volcano
- •shingle
- •shoal
- •shoaling
- •shock normal
- •shock spike
- •shock wave
- •shoreline
- •short wave fadeout (SWF)
- •sidereal day
- •sidereal month
- •sidereal time
- •sidereal year
- •siderophile
- •sieve analysis
- •Sievert
- •signature
- •sign convention
- •silent earthquake
- •silent universe
- •silicon burning
- •silt
- •Simon moments (1984)
- •simple harmonic motion
- •simple harmonic wave
- •simple pendulum
- •simultaneity, in Newtonian mechanics
- •single scattering albedo
- •single scattering phase function
- •singularities
- •singularity theorems
- •sinistral fault
- •Sinope
- •sinusoidal wave
- •Sirius
- •SI (Systeme International)
- •skewness
- •skip fading
- •skip zone
- •Skylab
- •slab model
- •slab penetration
- •slip system
- •slough
- •slow earthquake
- •slow magnetohydrodynamic shock
- •slow shock wave
- •slow solar wind
- •small amplitude wave
- •Small Magellanic Cloud (SMC,NGC 292)
- •small-scale turbulence
- •SNC meteorites
- •snow
- •soft gamma repeaters
- •Soft X-ray Telescope (SXT)
- •solar abundance
- •solar activity
- •Solar and Heliospheric Observatory (SOHO)
- •solar atmosphere
- •solar constant
- •solar cycle
- •solar day
- •solar disk
- •solar dynamo
- •solar eclipse
- •solar electromagnetic radiation
- •solar granulation
- •solar limb
- •solar magnetic sectors
- •solar maximum
- •solar maximum mission (SMM)
- •solar minimum
- •solar nebula
- •solar neutrinos
- •solar P-angle
- •solar quiet current system
- •solar radiation
- •solar rotation
- •solar spectral irradiance
- •solar system
- •solar system formation
- •solar wind
- •solar year
- •solar zenith angle
- •solid Earth tides
- •solitary wave
- •soliton
- •solstice
- •sound wave
- •source functions
- •source surface
- •South Atlantic Anomaly
- •South Equatorial current
- •southern oscillation
- •Southern Oscillation Index (SOI)
- •spacetime
- •space weather
- •spallation
- •special relativity
- •spectral
- •spectral energy distribution
- •spectral energy distribution of active galactic nuclei
- •spectral gap
- •spectral line
- •spectral type
- •spectrograph
- •spectrometer
- •spectrophotometry
- •spectropolarimetry
- •spectroradiometer
- •spectroscopic binary
- •spectroscopy
- •spectrum
- •spectrum binary
- •speed of light
- •spherical harmonic analysis
- •spherical pendulum
- •Spica
- •spicule
- •spinel
- •spinor
- •spin up
- •spiral arm
- •spiral galaxy
- •spontaneous symmetry breaking
- •sporadic E
- •spread E
- •spread F
- •spring
- •spring tide
- •sprites
- •squall
- •s (slow) process
- •S stars
- •stability frequency
- •stability of the water column
- •stability ratio
- •stable auroral red (SAR) arcs
- •stable causality
- •stable equilibrium
- •standard atmosphere
- •standard candle
- •standard pressure
- •standard project hurricane
- •standard temperature and pressure
- •standard time
- •standing shock
- •standing wave
- •Stanton number (
- •starburst galaxy
- •Stardust
- •star formation
- •star formation rate (SFR)
- •Stark effect
- •star spots
- •statcoulomb
- •state parameter
- •steepness (wave)
- •stellar activity
- •stellar evolution
- •stellar population
- •stellar winds
- •stepped leader
- •steric height
- •stick-slip
- •still water level
- •stishovite
- •stochastic acceleration
- •Stokes parameters
- •Stokes parameters
- •Stokes polarimetry
- •Stokes wave theory
- •Störmer orbits
- •Störmer theory
- •Störmer unit
- •storm sudden commencement (SSC)
- •storm surge
- •storm track
- •strain
- •strain hardening
- •strain partitioning
- •strain softening
- •stratosphere
- •stratovolcano
- •stratus
- •streakline
- •streamer belt
- •stream function wave theory
- •streamline
- •stress
- •stress drop
- •stress energy tensor
- •striations, auroral
- •string model
- •string network
- •stromatolite
- •strong anthropic principle
- •strong scattering limit
- •Strouhal number (
- •structure toe
- •St. Venant Equations
- •subduction
- •subduction zone
- •subdwarf
- •subgiant
- •subgrain size
- •submarine canyon
- •subsidence
- •subsolar point
- •subsonic string model
- •substorm injection
- •substorm phases
- •substorm triggering
- •substorm wedge
- •subtropical high-pressure belt
- •sudden frequency deviation (SFD)
- •sudden ionospheric disturbance (SID)
- •sudden phase anomaly (SPA)
- •sudden stratospheric warming
- •sulfur dioxide
- •summation convention
- •summer solstice
- •sunlit aurora
- •sunspot
- •sunspot cycle
- •sunspot number
- •sunspot number (daily)
- •sunspot number (smoothed)
- •sunward arc
- •supercell storm
- •super cloud cluster
- •supercluster
- •superconducting string
- •supercooling
- •supergeostrophic wind
- •supergiant
- •supergradient wind
- •supergranulation
- •superior conjunction
- •superior mirage
- •superluminal source
- •supermassive black hole
- •supernova
- •supernovae, 1987A
- •supernovae, 1991bg
- •supernovae, 1993J
- •supernovae, accretion induced collapse (AIC)
- •supernovae, core collapse mechanism
- •supernovae, delayed neutrino mechanism
- •supernovae, distance indicators
- •supernovae, expanding photosphere method
- •supernovae, fallback
- •supernovae, gravitational waves
- •supernovae, light curves
- •supernovae, neutrino detectors
- •supernovae, neutrino-driven wind mechanism
- •supernovae, neutrinos
- •supernovae, prompt shock mechanism
- •supernovae, spectra
- •supernovae, thermonuclear explosions
- •supernovae, Type Ia
- •supernovae, Type Ib/Ic
- •supernovae, Type II
- •supernovae, white dwarf accretion
- •supernova rates
- •supernova remnant (SNR)
- •Supernova Rates
- •superposition principle
- •supersonic
- •supersonic string model
- •superspace
- •surface boundary layer
- •surface gravity
- •surface tension (
- •surface waves
- •surf zone
- •surge
- •suspended load
- •suspension
- •suture
- •swash zone
- •swell
- •symbiotic star
- •symmetric instability
- •symmetry
- •synchronous orbit
- •synchronous rotation
- •synchrotron radiation
- •synchrotron self-Compton mechanism
- •synodical month
- •synodic month
- •synodic period
- •synoptic scale
- •synthetic aperture radar
- •syzygy
- •tachyon
- •tail current
- •Talwani’s method
- •tangential discontinuity
- •tangential geostrophy
- •Taygeta
- •Taylor instability
- •Taylor number
- •Taylor’s hypothesis
- •Taylor state
- •tectonics
- •tectosphere
- •Telesto
- •temperature
- •temperature inversion
- •temperature variance dissipation rate
- •tension (cosmic string)
- •tension head (ψ)
- •tensor
- •terminal velocity
- •terminator
- •Terrestrial Coordinate Time (TCG)
- •Terrestrial Dynamical Time (TDT)
- •terrestrial planets
- •Terrestrial Time (TT)
- •tesla
- •Tethys
- •Tethys Ocean
- •texture
- •Thalassa
- •Tharsis Province
- •Thebe
- •thermal anisotropy
- •thermal bar
- •thermal boundary layer
- •thermal bremsstrahlung
- •thermal conductivity
- •thermal diffusive sublayer
- •thermal diffusivity
- •thermal Doppler line broadening
- •thermal expansivity
- •thermal wind relationship
- •thermobaric effect
- •thermobaricity
- •thermocline
- •thermohaline circulation
- •thermosphere
- •Theta aurora
- •thick-target
- •thin-target
- •30 Doradus
- •Thompson circulation theorem
- •Thomson scattering
- •Thorpe displacement
- •Thorpe scale
- •thrust fault
- •Tibetan Plateau
- •tidal bore
- •tidal currents
- •tidal delta
- •tidal energy dissipation
- •tidal forces
- •tidal formation of solar system
- •tidal friction
- •tidal heating
- •tidal inlet
- •tidal period
- •tidal prism
- •tidal radius
- •tidal stripping
- •tidal tail
- •tide
- •tide range
- •tilt angle
- •time dilatation
- •time dilation
- •time-distance helioseismology
- •time in semiclassical gravity
- •time zone
- •Titan
- •Titania
- •Tolman model
- •tombolo
- •Tomimatsu–Sato metrics (1973)
- •Toomre’s stability parameter
- •TOPEX/POSEIDON
- •Top hat detector
- •topocentric system
- •topographic wave
- •topography
- •topological defect
- •topology of space
- •tornado
- •torque
- •torsion
- •torsional oscillation
- •total electron content (TEC)
- •total radiance mean cosine
- •towards polarity
- •tracers
- •traction
- •transcurrent (transform) plate boundary
- •transform boundary
- •transform fault
- •transform push
- •transient shock
- •transitional depth
- •transition region
- •translatory wave
- •transmissivity (
- •transonic string model
- •transparency
- •transpiration
- •transverse Doppler shift
- •transverse wave
- •trapped surface
- •traveling ionospheric disturbance (TID)
- •travel time curve
- •trench
- •trench excavation
- •triggered star formation
- •triple junction
- •triple point
- •tripton
- •tritium
- •trochoidal wave theory
- •Tritium Unit
- •Triton
- •Trojans
- •Trojans asteroids
- •tropical cyclone
- •tropical instability waves
- •tropical month
- •tropical storm
- •tropical year
- •Tropic of Cancer
- •Tropic of Capricorn
- •tropopause
- •troposphere
- •trough
- •true anomaly
- •true celestial equator
- •true celestial pole
- •true north
- •true polar wander
- •tsunami
- •tsunami earthquake
- •T Tauri object
- •T Tauri star
- •turbidity
- •turbulence
- •turbulence cascade
- •turbulent cascade
- •turbulent kinetic energy
- •twilight
- •twin paradox
- •twistor
- •2-form
- •two-stream approximation
- •two-stream instability
- •type I radio burst
- •type II radio burst
- •type III radio burst
- •type IV radio burst
- •typhoon
- •ultrasound
- •ultraviolet
- •Ulysses Mission
- •umbra
- •Umbriel
- •unconformities
- •undertow
- •unipolar magnetic region
- •unit weight
- •universal gravitation, Newton law of
- •Universal Time (UT or UT1)
- •universe
- •unstable equilibrium
- •upper mantle
- •upper neutral atmospheric regions
- •Uranus
- •Urca process
- •Urey ratio
- •V471 Tauri stars
- •vacuum
- •vacuum manifold
- •vacuum polarization
- •Väisälä frequency
- •Valles Marineris
- •valley networks
- •Van Allen Belts
- •VAN method
- •vapor
- •vapor concentration
- •vapor pressure
- •variable star
- •variable stars [cataclysmic variables]
- •variable stars [geometric variables]
- •variable stars [peculiar variables]
- •variable stars [pulsating variables]
- •variational principle
- •Vasyliunas theorem
- •vector
- •vector cross product
- •vector magnetograph
- •velocity
- •velocity curve
- •velocity dispersion
- •velocity distribution
- •velocity strengthening
- •velocity weakening
- •ventifacts
- •ventilated thermocline
- •Venturi tube
- •Venus
- •Venusian Tessera
- •vernal equinox
- •Very Long Baseline Interferometry (VLBI)
- •Vesta
- •virga
- •Virgo
- •Virgo constellation
- •Virgo Supercluster
- •virtual geomagnetic pole
- •viscoelastic material
- •viscosity
- •viscosity
- •viscous-like force
- •viscous shear stress
- •viscous sublayer
- •visible light
- •visible wavelengths
- •visual binary system
- •Vlasov equation
- •voids
- •Voigt body
- •volatile
- •volcanic front
- •volcanic line
- •volcanism
- •volcanos
- •volume scattering function (VSF)
- •volumetric water content
- •von Mises criterion
- •vortex defect of the vacuum
- •vortex street
- •vorticity
- •vorton
- •Wadati–Benioff zones (Benioff zones)
- •wake
- •wake (cosmic string)
- •Walker circulation
- •wall terracing
- •warm front
- •warping (of the neutral sheet or plasma sheet)
- •wash load
- •water
- •water mass
- •waterspout
- •water table
- •watt (W)
- •wave
- •wave-affected surface layer (WASL)
- •wave crest
- •wavefront
- •wave-function of the universe
- •wavelength
- •wave number
- •wave-particle interactions
- •wave rose
- •wave trough
- •wave vector
- •weak anthropic principle
- •weak interaction
- •weakly asymptotically simple space-time
- •weather
- •weather forecast
- •weight
- •weir
- •well-graded
- •Wesselink method
- •westerlies
- •western boundary currents
- •westward drift
- •wetness (or degree of saturation) (S)
- •wetted perimeter
- •Weyl space-times (1917)
- •Weyl tensor
- •whimper
- •whistlers
- •whistler waves
- •white dwarf
- •white hole
- •white light
- •Wien’s displacement law
- •Wien distribution law
- •Wien’s law
- •wiggle (cosmic string)
- •Wilson cycle
- •wind
- •wind chill factor
- •wind-driven circulation
- •wind evaporation-sea surface temperature feedback
- •winding number
- •wind rose
- •wind scale
- •wind shear
- •winter anomaly
- •winter solstice
- •Witten conducting string
- •wolf number
- •work
- •worldsheet charge
- •worldsheet geometry
- •wormhole
- •wrinkle ridges
- •W Ursa Majoris (W UMa)
- •WWSSN (World-Wide Standardized Seismograph Network)
- •xenoliths
- •xenon
- •X-ray source
- •yardangs
- •year
- •Yoshida jet
- •Yukawa coupling
- •Zeeman effect
- •Zeldovich process
- •zenith
- •zenith angle
- •zero mode
- •zodiac
- •zodiacal light
- •zonal
- •zooplankton
- •Zulu Time
- •Zwicky compact galaxies
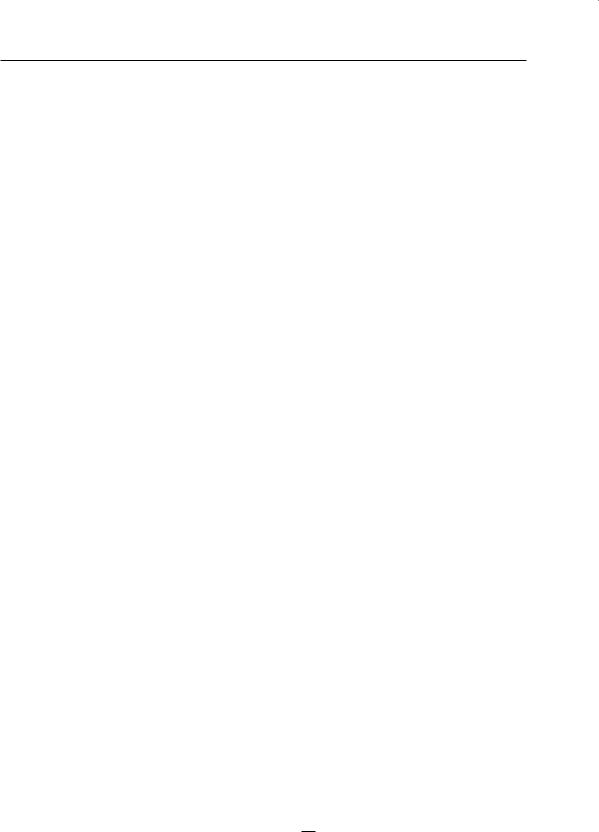
diffusion-convection equation
sionally, toward the second or higher diffraction orders. In this case, a grating is said to be blazed.
diffuse absorption coefficient For downwelling (upwelling) irradiance: the ratio of the absorption coefficient to the mean cosine of the downward (upward) radiance. See absorption coefficient.
diffuse attenuation coefficient For downwelling (upwelling) irradiance: the ratio of the sum of the absorption coefficient and the scattering coefficient to the mean cosine of the downward (upward) radiance.
diffuse aurora A weak diffuse glow of the upper atmosphere in the auroral zone, caused by collisions with the upper atmosphere of electrons with energies around 1 keV. It is believed that these electrons leak out from the plasma sheet of the magnetosphere, where they are trapped magnetically. The diffuse aurora is not conspicuous to the eye, but imagers aboard satellites in space see it as a “ring of fire” around the magnetic pole. Discovered by ISIS-1 in 1972, its size, intensity, and variations — in particular, its intensifications and motions in substorms — are important clues to the state of the magnetosphere.
diffuse galactic light The diffuse glow observed across the Milky Way. A large part of the brightness of the Milky Way, which is the disk of our galaxy seen from the inside, can be resolved into stellar sources. The diffuse galactic light is a truly diffuse glow which accounts for the remaining 25% of the luminosity and, by definition, is unresolved even if observed with large telescopes. The diffuse galactic light is due to light emitted within our galaxy and scattered by dust grains, and it is not to be confused with light coming from extended sources like reflection or emission nebulae. The brightness close to the galactic equator due to diffuse galactic light is equivalent to 50 stars of 10th magnitude per square degree; for comparison, the total star background is 170 10th magnitude stars per square degree, and the zodiacal light 80.
diffuse interstellar bands (DIBs) A series of interstellar absorption features recorded on
photographic plates in the early 1900s. They were labeled “diffuse” because they arise from electronic transitions in molecules, so they are broad in comparison to atomic lines. There are now well over 100 such bands known in the UV, visible, and near IR regions of the spectrum arising in interstellar clouds. DIBs must be molecular, given the complexity of the absorption lines. DIBs are easily seen when observing spectra of hot, fast rotating stars whose spectrum has a strong continuum. Even with very high resolution spectroscopy, the diffuse interstellar bands continue to show blended structures. DIBs show considerable scatter in strength vs. the amount of stellar reddening suggesting inhomogeneous variation of chemistry and dust-to-gas ratio. This may arise because the molecule(s) in the volume may be able to add hydrogen to the molecular structure in certain circumstances, as is known for some carbon compounds. Identifying the carriers of these absorptions has become perhaps the classic astrophysical spectroscopic problem of the 20th century, and numerous molecules have been put forth as the source of these features. Recently most attention has focused on carbon rich molecules such as fullerenes and polycyclic aromatic hydrocarbons.
diffuse scattering coefficient for downwelling (upwelling) irradiance The ratio of the scattering coefficient to the mean cosine of the downward (upward) radiance.
diffusion The gradual mixing of a quantity (commonly a pollutant) into a fluid by random molecular motions and turbulence.
diffusion-convection equation Transport equation for energetic charged particles in interplanetary space considering the effects of spatial diffusion and convection of particles with the solar wind. The transport equation can be derived from the equation of continuity by supplementing the streaming with the convective streaming vf , yielding
∂f + · (v sowif ) − · (D f ) = 0 ∂t
with f being the phase space density, D the (spatial) diffusion coefficient, and v sowi the solar
© 2001 by CRC Press LLC
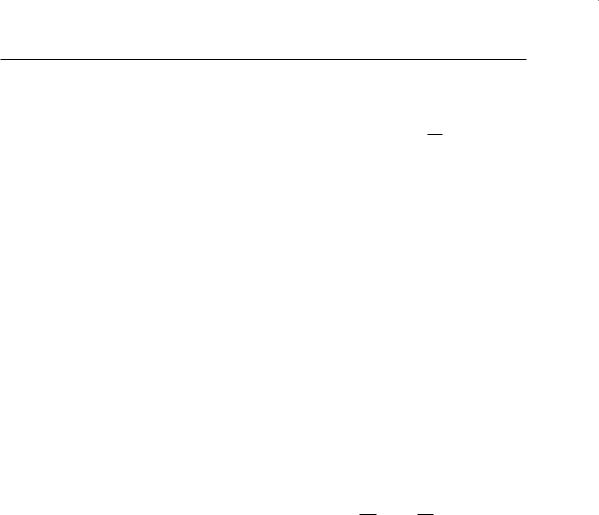
diffusion creep
wind speed. The terms then give the convection with the solar wind and spatial scattering. The diffusion-convection equation can be used to model the transport of galactic cosmic rays or the transport of solar energetic particles beyond the orbit of Earth.
If v and D are independent of the spatial coordinate, the solution of the diffusionconvection equation for a δ-injection in the radial-symmetric case, such as the explosive release of energetic particles in a solar flare, reads
|
= |
|
(4πDt)3 |
− |
4Dt |
|
f (r, t) |
|
|
N o |
exp |
(r − vt)2 |
. |
|
|
|
|
|||
|
|
|
|
|
|
This latter equation is a handy tool to estimate the particle mean free path from intensitytime profiles of solar energetic particle events observed in interplanetary space, although for careful studies of propagation conditions numerical solutions of the complete transport equation should be used.
diffusion creep When macroscopic strain is caused by diffusion transport of matter between surfaces of crystals differently oriented with respect to differential stress, it is called diffusion creep. Nabarro–Herring creep, where vacancies diffuse through the grain between areas of its boundary, and Coble creep, where diffusion takes place along the grain boundary, are two examples. Diffusion creep results dominantly from the motions (diffusion) of species and defects (vacancies and interstitial). It is characterized by (1) a linear dependence of strain rate on stress (n = 1, n is stress sensitivity of creep rate at steady-state stage); (2) high grain-size sensitivity; (3) the rate-controlling species is the lowest diffusion species along the fastest diffusion path; (4) no lattice preferred orientation formed;
(5) little transient creep; (6) deformation is stable and homogeneous.
diffusion, in momentum space Momentum transfer between particles can be due to collisions as well as due to wave-particle interaction. If these collisions/interactions lead to energy changes distributed stochastically, and the energy changes in individual collisions are small compared to the particle’s energy, the process can be described as diffusion in momentum
space. Instead of the particle flow considered in spatial diffusion, a streaming S p in momentum
results
∂f S p = −D pp ∂p
with p being the momentum, f the phase space density, and D pp the diffusion coefficient in momentum.
Also if non-diffusive changes in momentum, e.g., due to ionization, can happen, the streaming in momentum can be written as
S p = −D pp |
∂f |
+ |
dp |
|
|
|
f |
||
∂p |
dt |
with the second term corresponding to the convective term in spatial diffusion. See diffusion- convection equation.
diffusion, in pitch angle space Waveparticle interactions lead to changes in the particle’s pitch angle. When these changes are small and distributed stochastically, diffusion in pitchangle space results. The scattering term can be derived strictly analogous to the one in spatial diffusion by just replacing the spatial derivative by the derivative in pitch-angle µ:
∂∂f
κ(µ)
∂µ ∂µ
with f being the phase space density and κ(µ) being the pitch-angle diffusion coefficient. Note that κ depends on µ, that is scattering is different for different pitch-angles, depending on the waves available for wave-particle interaction. See resonance scattering, slab model.
diffusively stable A water column is called diffusively stable if both vertical gradients of salinity (β∂S/∂z) and of temperature (α∂?/∂z) enhance the stability N2 of the water column. The practical implication is that molecular diffusions of salt and temperature cannot produce local instabilities, as they can in double diffusion.
diffusive regime The diffusive regime is one of two possibilities, which allow double diffusion to occur. Under diffusive regimes, salinity stabilizes and temperature destabilizes the water column in such a way that the resulting vertical density profile is stable, i.e.,
© 2001 by CRC Press LLC
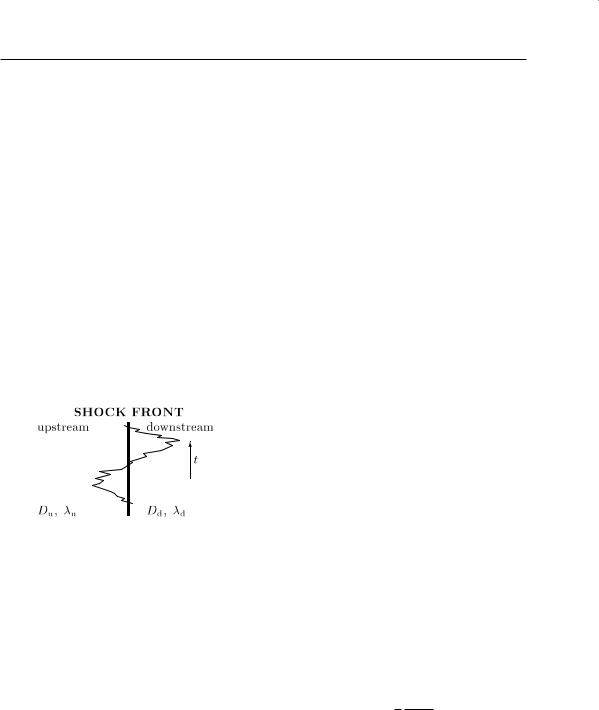
diffusive shock acceleration
Rρ =2 |
(β∂S/∂z)/(α∂?/∂z) > 1 (and stabil- |
|
ity N |
> 0). |
|
diffusive shock acceleration |
Acceleration |
due to repeated reflection of particles in the plasmas converging at the shock front, also called Fermi-acceleration. Diffusive shock acceleration is the dominant acceleration mechanism at quasi-parallel shocks because here the electric induction field in the shock front is small, and therefore shock drift acceleration is inefficient. In diffusive shock acceleration, the scattering on both sides of the shock front is the crucial process. This scattering occurs at scatter centers frozen-in into the plasma, thus particle scattering back and forth across the shock can be understood as repeated reflection between converging scattering centers (first order Fermi acceleration).
Particle trajectory in diffusive shock acceleration.
With f being the phase space density, U the plasma bulk speed, D the diffusion tensor, p the particle momentum, and T a loss time, the transport equation for diffusive shock acceleration can be written as
∂f |
|
U |
|
f |
|
|
(D |
|
f ) |
|
|
U |
p |
∂f |
|
f |
||
∂t |
+ |
|
− |
|
− |
|
|
+ T |
||||||||||
|
|
|
|
|
|
|
3 ∂p |
|||||||||||
+p2 |
|
∂p p2 |
dt f |
= Q(r, p, t) |
||||||||||||||
|
1 |
|
∂ |
|
|
|
|
dp |
|
|
|
|
|
|
|
|
with Q(r, p, t) describing an injection into the acceleration process. The terms from left to right give the convection of particles with the plasma flow, spatial diffusion, diffusion in momentum space (acceleration), losses due to particle escape from the acceleration site, and convection in momentum space due to processes that affect all particles, such as ionization or Coulomb losses.
In a first-order approximation, the last two terms on the right-hand side (losses from the acceleration site and convection in momentum space) can be neglected. In addition, if we limit ourselves to steady state, some predictions can be made from this equation:
1. Characteristic acceleration time. With the indices u and d denoting the properties of the upstream and downstream medium, the time required to accelerate particles from momentum po to p can be written as
t = uu |
3 |
|
p |
p |
· |
u u |
+ u d . |
||||
ud |
|||||||||||
|
|
|
|
dp |
|
D u |
|
Dd |
|||
|
|
− |
|
po |
|
|
|
|
|
|
|
Here D denotes the diffusion coefficient. Alternatively, a characteristic acceleration time τ a can be given as
τ a = |
3r |
|
|
D u |
r − |
1 |
|
u2u |
with r = u u/ud being the ratio of the flow speeds in the shock rest frame. For a parallel shock, r equals the compression ratio. τ a then gives the time the shock needs to increase the particle momentum by a factor of e. Note that here the properties of the downstream medium have been neglected: It is tacitly assumed that the passage of the shock has created so much turbulence in the downstream medium that scattering is very strong and therefore the term D d/ud is small compared to the term D u/u u.
2. Energy spectrum. In steady state, diffusive shock acceleration leads to a power law spectrum in energy J (E) = Jo · E−γ . Here the spectral index γ depends on the ratio r = u u/ud of the flow speeds only:
γ= 1 r + 2 2 r − 1
in the non-relativistic case, or γ rel = 2γ in the relativistic case.
3. Intensity increase upstream of the shock.
The spatial variation of the intensity around the shock front can be described as
f (x, p) = f (x, 0) exp{−β|x|}
with β = u u/D u. If β is spatially constant, an exponential intensity increase towards the shock
© 2001 by CRC Press LLC

dike
results. Because the particle mean free path λ increases with energy, the ramp is steeper for lower energies than for higher ones. In addition, the intensity at the shock front is higher for lower energies, reflecting the power-law spectrum. In the study of particle events, the upstream intensity increase is often used to determine the scattering conditions upstream of the shock.
4. Self-generated turbulence. A crucial parameter for the acceleration time is the strength of the interplanetary scattering, as can be seen from the equation for the acceleration time. Downstream of the shock turbulence is high because the disturbance shock has just passed by. Thus upstream scattering is the limiting factor. For typical conditions in interplanetary space, the Fermi process would develop too slowly to reach MeV energies during the time it takes the shock to travel from the sun to Earth. Nonetheless, these particles are observed. It is assumed that selfgenerated turbulence allows for more efficient scattering in the plasma upstream of the shock: at first, particles are accelerated to low energies only. As these particles propagate away from the shock, they generate and amplify Alfvén waves in resonance with the field parallel motion of the particles. These waves grow in response to the intensity gradient of the energetic particles and scatter particles back to the shock. These particles therefore interact again with the shock, gaining higher energy and, as they stream away from the shock front, generating waves with longer wavelength. This process repeats itself with the faster particles, and as acceleration on the shock continues, the particles acquire higher and higher energies and a turbulent region develops upstream of the shock. Such turbulent foreshock regions have been observed at traveling interplanetary shocks (proton energies up to some 100 keV and the waves in resonance with these particles) and at the quasi-parallel portion of the terrestrial bow shock (proton energies up to some 10 keV and waves in resonance with these particles). See resonance scattering.
dike A crack through which magma flows, the magma subsequently solidifying to form a thin planar igneous body.
dilatancy model A model to explain processes of earthquake generation, connecting with phenomena of anelastic volumetric expansion of rocks (dilatancy). At the beginning of the 1970s, C.H. Scholz proposed the model, dividing processes from strain accumulation to generation of a large earthquake into five stages. According to the dilatancy model, with increase of underground stresses, many cracks are formed, and pore water flows into the cracks. Then, pore pressure decreases, causing dilatancy hardening. Subsequently, the pore pressure gradually increases due to water supply from the ambient region, reaching main rupture. Land uplift preceding a large earthquake, temporal change in P-wave velocity, advent of seismic gap, and activity of foreshocks might be better explained by the model. However, since actual earthquakes take place on planes with mechanical defects in the crust, dilatancy does not necessarily develop sufficiently to explain a large earthquake. Reliability of observation for temporal change in P-wave velocity is also suspect. Therefore, the dilatancy model has attracted little attention in recent years.
dilatation of time-Lorentz transformation
The increase in the time interval of an event when measured in a uniformly moving reference system rather than in the reference system of the event, as calculated by the Lorentz Transformations in the Special Theory of Relativity. In special relativity time is not an absolute variable, and it therefore varies for different reference systems. See also coordinate transformation in special relativity. See time dilatation.
dilaton A scalar component of gravity which emerges in the low energy limit of string theory. See dilaton gravity.
dilaton gravity In the framework of string theory the field equations of general relativity are obtained as an approximation which is valid only for distances larger than the typical (microscopic) string length (low energy). Further, since string theory is a theory of extended objects (including p-dimensional branes, with p ≥ 2 and integer), one expects to have (nonlocal) corrections to Einstein’s field equations. The simplest corrections are extra fields, among
© 2001 by CRC Press LLC