
- •PREFACE
- •CONTRIBUTORS
- •Abbott, David C.
- •Abelian Higgs model
- •Abelian string
- •aberration of stellar light
- •Abney’s law of additivity
- •A-boundary
- •absolute humidity
- •absolute magnitude
- •absolute viscosity
- •absolute zero
- •absorbance
- •absorptance
- •absorption cross-section
- •absorption fading
- •absorption line
- •abstract index notation
- •abyssal circulation
- •abyssal plain
- •acceleration
- •acceleration due to gravity (g)
- •accreted terrain
- •accretion
- •accretionary prism (accretionary wedge)
- •accretion disk
- •accretion, Eddington
- •accretion, hypercritical
- •accretion, Super-Eddington
- •Achilles
- •achondrite
- •achromatic objective
- •achronal set
- •acoustic tomography
- •actinides
- •action
- •activation entropy
- •activation volume
- •active continental margin
- •active fault
- •active front
- •active galactic nuclei (AGN)
- •active margins
- •active region
- •adiabat
- •adiabatic atmosphere
- •adiabatic condensation point
- •adiabatic cooling
- •adiabatic deceleration
- •adiabatic dislocation
- •adiabatic equilibrium
- •adiabatic index
- •adiabatic invariant
- •adiabatic lapse rate
- •ADM form of the Einstein–Hilbert action
- •ADM mass
- •Adrastea
- •advance of the perihelion
- •advection
- •advection dominated accretion disks
- •advective heat transfer (or advective heat transport)
- •aeolian
- •aerosol
- •aesthenosphere
- •African waves
- •afternoon cloud (Mars)
- •aftershocks
- •agonic line
- •airfoil probe
- •airglow
- •Airy compensation
- •Airy isostasy
- •Airy phase
- •Airy wave theory
- •Aitken, John
- •Aitken nucleus count
- •Alba Patera
- •albedo
- •albedo neutrons
- •albedo of a surface
- •albedo of single scattering
- •Alcyone
- •Aldebaran
- •Alfvénicity
- •Alfvén layer
- •Alfvén shock
- •Alfvén speed
- •Alfvén’s theorem
- •Alfvén wave
- •Algol system
- •Allan Hills meteorite
- •allowed orbits
- •all sky camera
- •alluvial
- •alluvial fan
- •alongshore sediment transport
- •Alpha Centauri
- •alpha particle
- •Altair
- •alternate depths
- •altitude
- •Amalthea
- •Amazonian
- •Ambartsumian, Viktor Amazaspovich
- •Amor asteroid
- •ampere
- •Ampere’s law
- •amphidrome (amphidromic point)
- •Am star
- •anabatic wind
- •analemma
- •Ananke
- •Andromeda galaxy
- •anelastic deformation
- •anemometer
- •angle of repose
- •Ångström (Å)
- •angular diameter distance
- •angular momentum L
- •angular velocity (
- •anisotropic
- •anisotropic scattering
- •anisotropic turbulence
- •anisotropic universe
- •anisotropy
- •annular eclipse
- •anomalistic month
- •anomalistic year
- •anomalous resistivity
- •anomaly
- •anomaly, South Atlantic
- •anorthosite
- •anoxia
- •Antarctic circle
- •antarctic circumpolar current
- •Antarctic ozone depletion
- •Antarctic ozone hole
- •Antarctic Zone
- •Antares
- •anthropic principle
- •anticyclone
- •anticyclonic
- •antidune
- •antinode
- •antiparticle
- •apastron
- •aperture correction
- •aperture synthesis
- •aphelion
- •aphotic zone
- •Ap index
- •apoapsis
- •Apollo asteroid
- •apparent horizon
- •apparent magnitude
- •apparent optical property (AOP)
- •apparent solar time
- •apse
- •Ap star
- •aquifer
- •aquitard
- •Arago point
- •arcade
- •archaeoastronomy
- •archaeomagnetism
- •Archean
- •Archimedes’ principle
- •Archimedian spiral
- •arc minute
- •arc second
- •Arctic circle
- •arctic oscillation (AO)
- •Arcturus
- •argon
- •argument of periapse
- •Ariel
- •array seismic observation
- •arrow of time
- •ascending node
- •aseismic front
- •aseismic region
- •asperity
- •association
- •asterism
- •asteroid
- •Asterope
- •asthenosphere
- •Astraea
- •astrochemistry
- •astrometric binary
- •astrometry
- •astronomical latitude
- •astronomical refraction
- •astronomical scintillation
- •astronomical tide
- •astronomical twilight
- •astronomical unit (AU)
- •astronomy, infrared
- •astronomy, ultraviolet: interstellar
- •asymmetry factor
- •asymmetry parameter
- •asymptotic
- •asymptotically simple space-time
- •asymptotic giant branch (AGB) star
- •asymptotic regime
- •Aten asteroid
- •Atlas
- •atmosphere
- •atmosphere effect
- •atmospheric angular momentum
- •atmospheric conductivity
- •atmospheric noise
- •atmospheric pressure
- •atmospherics
- •atmospheric tide
- •atomic mass
- •atomic number
- •atomic structure calculations — one-electron models
- •atomic time
- •aulacogen
- •aurora
- •aurora australis
- •aurora borealis
- •auroral cavity
- •auroral electrojet
- •auroral oval
- •auroral zone
- •autumnal equinox
- •available potential energy
- •avalanche
- •average cosine
- •averaging
- •Avogadro’s number
- •away polarity
- •AXAF
- •axial dipole principle
- •axionic string
- •azimuth
- •Baade–Wesselink method
- •Babinet point
- •baby universe
- •backarc spreading
- •back scattering
- •backscattering fraction
- •backshore
- •Backus effect
- •Baily’s beads
- •Ballerina model
- •Balmer series
- •banner cloud
- •bar detectors
- •barium release
- •Barnard’s star
- •baroclinic atmosphere or ocean
- •baroclinic instability
- •baroclinic wave
- •barotropic atmosphere or ocean
- •barotropic instability
- •barotropy
- •barred galaxies
- •barrier island
- •barriers
- •Barycentric Dynamical Time (TDB)
- •baryogenesis
- •basalt
- •basaltic lava
- •basement
- •basic MUF
- •Batchelor scale
- •Batchelor spectrum
- •Batchelor wavenumber
- •batholith
- •bathymetry
- •b-boundary
- •beach cusps
- •beachface
- •beach mining
- •beach morphology
- •beach nourishment
- •beach ridge
- •beams, ion
- •beam spread function
- •beam transmissometer
- •Beaufort wind scale
- •bed load
- •Beer’s law
- •Belinda
- •Benard cell
- •Benioff zone
- •benthic
- •benthos
- •Bergen school
- •Bergeron, Tor
- •berm
- •Bernoulli equation
- •Besselian year
- •beta decay
- •beta-effect
- •Beaufort Wind Scale Limits of Wind Speed at 10 m
- •beta-plane approximation
- •Bianca
- •biased vacuum states (domain wall)
- •bias frame, bias overscan
- •biasing parameter
- •Big Bang
- •Big Bang cosmology
- •Big Bang horizon problem
- •Big Bang nucleosynthesis
- •big blue bump
- •binary black holes
- •binary fraction
- •binary pulsar
- •binary star
- •binary star system
- •bioluminescence
- •biomass
- •biosphere
- •BIPM
- •Birkeland current
- •Birkhoff theorem (1923)
- •Bjerknes
- •Bjerknes circulation theorem
- •Bjerknes feedback
- •black aurora
- •black-body radiation
- •blackbody temperature
- •black frost
- •black hole
- •black hole binary
- •black hole horizon
- •black ice
- •blast wave shock
- •blazar
- •blizzard
- •BL Lacertae
- •BL Lacertae object
- •blocking
- •blocking patterns
- •blue clearing (Mars)
- •blue ice
- •blue jet
- •blue straggler
- •body waves
- •Bogomol’nyi bound
- •Bohr’s theory of atomic structure
- •bolide
- •bolometric correction (B.C.)
- •bolometric magnitude/luminosity
- •Boltzmann’s constant (
- •Bondi mass
- •Bonnor symmetry
- •bore
- •borrow site
- •boson
- •boson star
- •Bouguer correction
- •Bouguer gravity anomaly
- •bounce motion
- •boundary conditions
- •boundary layer pumping
- •Boussinesq approximation
- •Boussinesq assumption
- •Boussinesq equation
- •Bowen’s ratio
- •Boyle’s law
- •Brackett series
- •Bragg angle
- •Bragg Crystal Spectrometer (BCS)
- •braided river
- •Brans–Dicke theory
- •Brazil current
- •breaker zone
- •breakwater
- •breccias
- •Bremsstrahlung
- •bremstrahlung [thermal]
- •Brewster point
- •brightness
- •brightness temperature
- •bright point
- •brittle behavior (brittle fracture)
- •brittle-ductile transition
- •brittle-plastic transition
- •broad line radio galaxies
- •broad line region
- •brown dwarf
- •Brunt frequency
- •Brunt–Väisälä frequency
- •B star
- •bulkhead
- •bulk modulus
- •bulk parameters
- •buoyancy
- •buoyancy frequency
- •buoyancy Reynolds number
- •buoyancy scale
- •buoyancy scaling
- •buoyancy subrange
- •Bureau International des Poids et Mesures (BIPM)
- •Burgers vector (b)
- •burst
- •Buys Ballots Law
- •b-value
- •Byerlee’s law
- •bypass
- •cabbeling
- •cable
- •caldera
- •calendar
- •California current
- •California nebula
- •Callan–Rubakov effect
- •Callisto
- •calorie
- •Calypso
- •Canary current
- •candela
- •canonical momentum
- •canonical transformation
- •Canopus
- •cap cloud
- •CAPE (Convective Available Potential Energy)
- •Capella
- •capillarity correction
- •capillary fringe
- •capillary wave
- •carbon-14 dating
- •carbonaceous chondrite
- •carbon burning
- •carbon cycle
- •carbon dioxide
- •carbon monoxide
- •carbon star
- •Caribbean current
- •Carme
- •Carnot cycle
- •Carnot engine
- •Carrington longitude
- •Carrington rotation
- •Cartesian coordinates
- •Cartesian coordinates [in a plane]
- •Cartesian coordinates [in space]
- •case 1 water
- •case 2 water
- •Cassegrainian
- •Cassini
- •Cassini’s division
- •Cassiopeia A
- •Castor
- •Castor, John I.
- •cataclysmic variable (cataclysmic binary)
- •cataclysmic variable [binary models of]
- •cataclysmic variable [galactic distribution]
- •cataclysmic variable [outbursts]
- •cataclysmic variables [physical parameters]
- •catastrophic formation of solar system
- •Cauchy singularity
- •causal boundary
- •causal curve
- •causal future/past
- •causality relations
- •cavity, magnetic
- •cD galaxies
- •Celaeno
- •celerity
- •celerity
- •celestial equator
- •celestial poles
- •celestial sphere
- •Celsius, Anders
- •Celsius scale
- •Centaur
- •Centaurus A (Cen A)
- •center of mass
- •Centigrade scale
- •centroid moment tensor (CMT)
- •centimeter burst
- •central meridian passage
- •central peak
- •central pit
- •centrifugal force
- •Cepheid variable
- •Cerenkov radiation
- •Ceres
- •CGS (Centimeter-Gram-Second)
- •chalcophile
- •Challenger Deep
- •Chandler wobble
- •Chandrasekhar limit (or Chandrasekhar mass)
- •chaos
- •chaotic cosmology
- •chaotic system
- •chaotic terrain
- •Chapman layer
- •characteristic earthquake
- •characteristics, method of
- •charge exchange
- •Charles’ Law
- •Charon
- •chemically peculiar star
- •chenier
- •chlorinity
- •chlorophyll
- •chondrite
- •chondrite, carbonaceous
- •chondrite, ordinary
- •chondrule
- •Christoffel symbol
- •chromatic aberration
- •chromosphere
- •chromospheric evaporation
- •chromospheric heating
- •chronological future/past
- •CHUMP (Charged Hypothetical Ultra Massive Particle)
- •cigar distribution
- •circulation
- •cirrus
- •civil time
- •civil twilight
- •Clapeyron–Clausius equation
- •clapotis
- •classical radius of electron
- •clathrate
- •clay
- •clear air turbulence (CAT)
- •cleft, polar
- •climate regimes
- •climatic optimum
- •closed magnetosphere
- •closed universe
- •clump star
- •CNO cycle (or tricycle)
- •cnoidal wave
- •coastal jet
- •coastally trapped waves
- •coastal ocean
- •coastal upwelling or downwelling
- •Coastal Zone Color Scanner (CZCS)
- •cobble
- •coble creep (grain-boundary diffusion creep)
- •cobpoint
- •coda wave
- •coesite
- •cohesionless
- •cohesive sediment
- •cold dark matter
- •cold front
- •cold plasma
- •Coleman–Weinberg potential
- •collision boundary
- •collisionless shock
- •colored dissolved organic matter (CDOM)
- •color excess
- •color index
- •color-magnitude diagram
- •color temperature
- •coma
- •comet
- •comet(s): chemical composition of
- •comet(s): dirty iceball or snowball model
- •comet(s): missions to
- •comminution
- •common envelope
- •common envelope binary
- •comoving frame
- •compact group of galaxies
- •compaction
- •compact steep spectrum radio sources
- •compensation
- •compensation depth
- •composite volcanos
- •compound channel
- •compressibility
- •Compton, Arthur H.
- •Compton cooling
- •Compton scattering
- •computational relativity
- •conditional unstability
- •conducting string
- •conduction
- •conductive heat transfer
- •conductivity of water
- •conformal tensor
- •congruence
- •conical spacetime
- •conics
- •conic section
- •conjugate depths
- •conjunction
- •connection
- •connection longitude
- •Conrad discontinuity
- •conservation of angular momentum
- •conservation of energy
- •conservation of momentum
- •conservative system
- •constellation
- •constitutive law of frictional sliding
- •constraint equations
- •contact binary
- •continent
- •continental climate
- •continental collision
- •continental drift
- •continental margin
- •continental plate
- •continental shelf
- •continental shelf waves
- •continuum
- •contravariant vector
- •convection
- •convection zone
- •convective adjustment
- •convective cloud
- •convective heat transfer
- •convective instability
- •convective scaling
- •convective turbulence
- •convergent boundary
- •convergent plate boundaries
- •Coordinated Universal Time (UTC)
- •coordinate singularity
- •coordinate system
- •coordinate time
- •coordinate transformation in special relativity
- •Copernicus, Nicholas
- •coplanarity theorem
- •Cordelia
- •cordillera
- •core
- •core collapse
- •core convection
- •core-dominated quasars
- •core-mantle coupling
- •Coriolis
- •Coriolis effect (Coriolis force)
- •Coriolis, Gaspard Gustave de
- •Coriolis parameter
- •corner frequency
- •corona
- •coronagraph
- •Coronal Diagnostic Spectrometer (CDS)
- •coronal dimming
- •coronal heating
- •coronal hole
- •coronal lines
- •coronal loops
- •coronal mass ejection (CME)
- •coronal rain
- •coronal transients
- •coronal trap
- •corotating interaction region
- •correlation length
- •coseismic deformation
- •cosine collector
- •cosmic abundance
- •cosmic censorship
- •cosmic microwave background
- •cosmic microwave background, spectral distortions
- •cosmic nucleosynthesis
- •cosmic phase transition
- •cosmic rays
- •cosmic spring
- •cosmic string
- •cosmic texture
- •cosmic topological defect
- •cosmochemistry
- •cosmogenic nuclides
- •cosmological constant
- •cosmological constant problem
- •cosmological model
- •cosmological principle
- •cosmology
- •coude focus
- •coulomb
- •Coulomb collisions
- •Coulter counter
- •Courant number
- •covariant derivative
- •covariant vector
- •Cox number
- •CP problem
- •Crab Nebula
- •Crab Pulsar
- •CRAND (Cosmic Ray Albedo Neutron Decay)
- •crater
- •crater depth-diameter plots
- •cratering rates
- •cratering record
- •crater number: index of age
- •crater production function
- •crater saturation
- •craton
- •creep
- •Cressida
- •critical current (cosmic string)
- •critical density
- •critical depth
- •critical frequency
- •critical level
- •critical phenomena in gravitational collapse
- •critical point
- •critical temperature
- •critical velocity
- •cross helicity
- •cross-section
- •cross-shore
- •cross slip
- •cross waves
- •crust
- •crustal deformation
- •crustal movement
- •crystal
- •crystallization age
- •CTRS
- •cumulative size-frequency curves
- •cumulus
- •Curie (Ci)
- •Curie point
- •Curie point survey
- •curl
- •current carrier (cosmic string)
- •current, curvature
- •current generation (cosmic string)
- •current, gradient
- •current instability (cosmic string)
- •current, magnetization
- •current, polarization
- •current quenching
- •current screening (cosmic string)
- •current sheet
- •curvature
- •curvature invariant
- •curvature tensor
- •curve
- •curved space-time
- •curve of growth
- •curvilinear coordinates
- •curvilinear coordinates [in a plane]
- •curvilinear coordinates [in space]
- •cusp (cosmic string)
- •cusp, polar
- •cutoff energy
- •cyclic coordinate
- •cyclone
- •cyclongenesis
- •cyclotron damping and instability
- •cyclotron frequency
- •cyclotron radius
- •Cygnus A
- •Cygnus Loop
- •cynthion
- •Cytherean
- •Dalton’s Law
- •Darcy’s law
- •Darcy–Weisbach friction factor
- •dark cloud
- •dark matter
- •dark matter, cold
- •dark matter, hot
- •dark nebula
- •dart leader
- •data assimulation
- •Davidson current
- •daylight savings time
- •dead zone
- •Deep Space 1 (DS1)
- •Deep Space 2
- •Deep Space Network (DSN)
- •deep water wave
- •defect
- •deferent
- •deformation radius
- •degeneracy
- •degree (temperature)
- •de Hoffman–Teller frame
- •Deimos
- •delta
- •Delta Scuti stars
- •Delta surface approximation
- •density
- •density current
- •density inversion
- •depleted mantle
- •depletion layer
- •depth of compensation
- •Derrick theorem
- •Debye length
- •decelleration parameter
- •decibel
- •declination
- •decollement
- •deep(-focus) earthquake
- •Descartes ray
- •Desdemona
- •deSitter Universe
- •Despina
- •detritus
- •deuterium
- •deuterium burning
- •de Vaucouleurs’ law
- •deviative absorption
- •deviatoric strain
- •deviatoric stress
- •dew point
- •dextral fault
- •diapir
- •diapycnal
- •dichotomy of Mars
- •diel
- •dielectric strength
- •differential charging
- •differential diffusion
- •differential emission measure
- •differential heating/cooling
- •differential rotation
- •differentiation
- •diffraction
- •diffraction grating
- •diffuse aurora
- •diffuse galactic light
- •diffuse interstellar bands (DIBs)
- •diffusion
- •diffusion-convection equation
- •diffusion creep
- •diffusion, in momentum space
- •diffusion, in pitch angle space
- •diffusively stable
- •diffusive regime
- •diffusive shock acceleration
- •dike
- •dilatancy model
- •dilatation of time-Lorentz transformation
- •dilaton
- •dilaton gravity
- •dilatonic black hole
- •dilution
- •dimension
- •dimensional analysis
- •dimensional transmutation
- •Dione
- •dip-equator
- •dip, magnetic
- •dip slip fault
- •disconnection event
- •discordant redshift
- •discrete aurora
- •discrimination (seismic)
- •disk warp
- •dislocation climb
- •dislocation creep
- •dislocation energy (self-energy of dislocation)
- •dislocation glide
- •disparition brusque
- •dispersion
- •dispersionless injection
- •dispersion measure (DM)
- •dispersive
- •displacement vector
- •dissipation
- •dissipation of temperature variance
- •dissipation of turbulent kinetic energy
- •dissipation range
- •dissipation rate
- •dissolved organic matter
- •distance indicator
- •distance modulus
- •diurnal
- •diurnal motion
- •divergence
- •divergence law for irradiance
- •divergence theorem
- •divergent boundary
- •divergent plate boundary
- •diversity reception
- •divided bar
- •D-layer
- •dog days
- •doldrums
- •domain of dependence
- •domain of outer communication
- •domain wall
- •dominant energy condition
- •Doodson number
- •Doppler beaming
- •Doppler broadening
- •Doppler dimming
- •Doppler effect
- •Doppler shift
- •double couple
- •double diffusion
- •double probe
- •double star
- •downward (upward) radiance mean cosine
- •downwelling
- •draconitic month
- •dragging phenomenon
- •dredge
- •D region
- •drift
- •drift, curvature
- •drift, electric
- •drift, gradient
- •drift, magnetization
- •driftmeter
- •drift, polarization
- •driven shock
- •drizzle
- •drumlins
- •drunkards walk
- •dry-adiabatic lapse
- •dry freeze
- •duality in elastic string models
- •ductile behavior
- •dune
- •dusk
- •dust
- •dust storm
- •dust storm (Mars)
- •dwarf galaxy
- •dwarf nova
- •dwarf spheroidal galaxies
- •dynamical friction
- •dynamical time (DT)
- •dynamic height
- •dynamic meter
- •dynamic pressure
- •dynamic recrystallization
- •dynamics
- •dynamic viscosity
- •dynamo
- •Eady, E.T.
- •Eady model
- •Earth
- •earth orientation parameters (EOP)
- •earthquake
- •earthquake intensity
- •earthquake magnitude
- •earthquake moment
- •earthquake precursor
- •earthquake prediction
- •earthquake swarm
- •earth rotation parameters (ERP)
- •earthshine
- •East Australian current
- •East Greenland current
- •easy access region
- •ebb current
- •ebb delta
- •ebb shoal
- •ebb tide
- •eccentric
- •eccentric dipole
- •eccentricity
- •echelle spectrograph
- •echo sounder
- •eclipse
- •eclipse year
- •eclipsing binary
- •ecliptic
- •Eddington approximation
- •Eddington limit
- •Eddington luminosity
- •Eddington ratio
- •eddy
- •eddy correlation method
- •eddy diffusivity
- •eddy-resolving
- •eddy viscosity
- •edge wave
- •effective charge
- •effective couplings
- •effective pressure
- •effective stress
- •effective temperature
- •eigenray
- •eigenvalue
- •eikonal approximation
- •einstein
- •Einstein equations
- •Einstein summation convention
- •Einstein tensor
- •Einstein Universe
- •ejecta
- •Ekman convergence
- •Ekman layer
- •Ekman mass transport
- •Ekman pumping
- •Elara
- •elastic deformation
- •elastic limit
- •elastic lithosphere
- •elastic modulus
- •elastic rebound
- •elastic string model
- •E-layer screening
- •Electra
- •electric drift
- •electric regime (cosmic string)
- •electroglow
- •electromagnetic current meter
- •electromagnetic induction
- •electromagnetic radiation
- •electromagnetism
- •electron
- •electron precipitation
- •electron temperature
- •electron volt
- •electrostatic unit
- •electrovacuum
- •element
- •elemental abundances: general
- •elemental abundances: in minerals
- •elemental abundances: in organics
- •elemental abundances: of comet(s)
- •elevation
- •elliptical galaxies
- •Ellison scale
- •elongation
- •elongation
- •elves
- •Elysium Province
- •embedded defect
- •emission line
- •emission lines: interstellar and cometary
- •emissivity
- •empirical model of the magnetosphere
- •Enceladus
- •endothermic
- •energetic particles
- •energetic particles in interplanetary space
- •Energetic Particle Population in the Heliosphere
- •energetic storm particles
- •energy
- •energy-containing scale
- •energy grade line
- •energy per unit length (cosmic string)
- •enthalpy
- •entrainment
- •entropy
- •environmental lapse rate
- •eolian
- •Eötvös experiment
- •epeiric sea
- •ephemeris
- •ephemeris time (ET)
- •epicenter
- •epicentral distance
- •epicycle
- •Epimetheus
- •epoch
- •equant
- •equation of continuity
- •equation of state
- •equation of state (cosmic string)
- •equation of state of pure water
- •equation of time
- •equator
- •equator, geomagnetic
- •equatorial anomaly
- •equatorial bulge
- •equatorial cold tongue
- •equatorial convergence zone
- •equatorial easterlies
- •equatorial electrojet
- •equatorial Kelvin wave
- •equatorial surface of the magnetosphere
- •equatorial undercurrent
- •equatorial upwelling
- •equatorial waveguide
- •equatorial waves
- •equilibrium
- •equilibrium range
- •equilibrium space-times
- •equilibrium tide (gravitational tide)
- •equilibrium vapor pressure
- •equinox
- •equivalence principle
- •Eratosthenes
- •E region
- •ergodic motion
- •ergoregion
- •ergs
- •Ernst equation (1967)
- •erosion
- •Ertel potential vorticity
- •eruption
- •eruptive prominence
- •escape velocity
- •eskers
- •estuary
- •eternal black hole
- •Eudoxos of Cnidus
- •Euler equations
- •Eulerian
- •Eulerian coordinates
- •Eulerian representation
- •Eulerian velocity
- •Euler, Leonhard
- •Euler pole
- •Euler potentials
- •euphotic depth
- •euphotic zone
- •euphotic zone midpoint
- •Europa
- •eutrophic water
- •evaporation (
- •evapotranspiration (
- •evening cloud (Mars)
- •event horizon
- •Evershed effect
- •evolutionary track
- •exact solution
- •excitation temperature
- •exosphere
- •exotic terrane
- •expansion
- •expansion of the universe
- •experimental craters
- •extended object
- •extensions of space-times
- •extinction and reddening
- •extratropical storm
- •extreme ultraviolet (EUV)
- •extreme ultraviolet imaging telescope (EIT)
- •eye (of a storm)
- •F1-layer
- •F2-layer
- •facula
- •fading
- •failed arm
- •faint young sun paradox
- •Falkland current
- •fallout
- •fall speed (velocity)
- •Fanaroff–Riley (FR) class I and II radio galaxies
- •Faraday Cup
- •Faraday effect
- •Faraday rotation
- •fast magnetohydrodynamic shock
- •fast shock wave
- •fast solar wind
- •Fata Morgana
- •fathom
- •fault
- •fault constitutive law
- •fault gouge
- •fault parameter
- •fault plane solution
- •fault scarp
- •F corona
- •feeder beach
- •feldspar
- •Fermat’s principle
- •fermion
- •fermionic zero mode
- •Ferrel cell
- •fertile mantle
- •fetch
- •FG Sagittae star
- •Fick’s second law
- •field line motion
- •finestructure
- •fjord
- •FK Comae stars
- •flocculation
- •Flora
- •Florida current
- •fluxrope
- •focal length
- •focal mechanisms
- •focus
- •focused transport equation
- •focusing
- •foehn
- •folds
- •following spot
- •Fomalhaut
- •forbidden lines
- •forbidden orbits
- •forbidden region
- •Forbush decrease
- •force
- •force balance
- •Forchhammer’s Principle
- •foreshore
- •foreshock
- •forecasting (wave)
- •forearc sliver
- •forearc basin
- •Foucault pendulum
- •four-velocity
- •fractal
- •fractionation
- •fracture
- •fracture zone
- •fragmentation
- •frame dragging
- •Fraunhofer lines
- •free-air gravity anomaly
- •free atmosphere
- •free bodies
- •free-bound continuum emission
- •free convection
- •free-free continuum emission
- •free oscillations
- •freeze
- •F region
- •Fresnel zone
- •fretted channels
- •fretted terrain
- •friction
- •friction factor
- •friction slope
- •friction velocity
- •Friedmann, Aleksandr Aleksandrovich
- •Friedmann–Lemaître cosmological models
- •fringe (interference)
- •front
- •frontogenesis
- •frost point
- •Froude number
- •F star
- •Fukushima’s theorem
- •fully arisen sea
- •fulvic substance
- •fundamental tensors of a worldsheet
- •future/past causal horizon
- •future/past event horizon
- •gabbro
- •galactic bulge
- •galactic cluster
- •galactic coordinates
- •galactic disk
- •galactic globular cluster
- •galactic noise
- •galactic wind
- •Galatea
- •galaxy
- •Galilean invariance
- •Galilean relativity
- •Galilean transformation
- •Galilei, Galileo
- •Galileo spacecraft
- •Galiliean satellite
- •gallium
- •gamma
- •gamma ray burst (GRB)
- •gamma-ray burst, black hole accretion disks
- •gamma-ray burst, classical
- •gamma-ray burst, cosmological mechanisms
- •gamma-ray burst, galactic mechanisms
- •gamma-ray burst, GRB 970508
- •gamma-ray burst, hypernova
- •gamma-ray burst, mechanisms
- •gamma-ray burst models, collapsar
- •gamma-ray burst models, helium merger
- •gamma-ray burst, soft gamma-ray repeaters
- •Ganymede
- •garden hose angle
- •gas constant
- •gaseous shocks
- •gas thermometer
- •gauge
- •gauge pressure
- •Gauss
- •gegenschein
- •gelbstoff
- •general circulation model (GCM)
- •general relativity
- •geocentric
- •geocentric coordinate time
- •geocentric latitude
- •geocorona
- •geodesic
- •geodesic completeness
- •geodesy
- •geodetic latitude
- •geodynamics
- •geodynamo
- •Geographus
- •geoid
- •geoid anomalies
- •geomagnetic activity
- •geomagnetic dip equator
- •geomagnetic disturbance
- •geomagnetic elements
- •geomagnetic indices
- •geomagnetic jerk
- •geomagnetic potential
- •geomagnetic storm
- •geometrodynamics
- •geophysics
- •geopotential
- •geopotential surface
- •geospace
- •geostrophic adjustment
- •geostrophic approximation
- •geostrophic balance
- •geostrophy
- •Geosynchronous Operational Environmental Satellites (GOES)
- •geosynchronous orbit
- •geotherm
- •geothermal energy
- •geothermal gradient
- •Geroch group
- •Gershun’s law
- •Gershun tube
- •giant branch
- •giant cells
- •giant planet
- •Gibbs free energy (Gibbs Potential)
- •gilvin
- •Ginzburg temperature
- •glacial
- •glaciation
- •glacier
- •global loop oscillation
- •globally hyperbolic space-time
- •global positioning system (GPS)
- •global seismology
- •global thermohaline circulation
- •global topological defect
- •globular cluster
- •gnomon
- •Goddard, Robert H.
- •Goldstone boson
- •Goldstone model
- •Goldstone theorem
- •Gondwanaland
- •graben
- •GRACE (Gravity Recovery and Climate Experiment)
- •gradient
- •gradient drift
- •gradient Richardson number
- •gradual commencement storm
- •grain-boundary migration
- •grain-boundary sliding
- •grain chemistry: dense interstellar
- •grain chemistry: diffuse interstellar
- •grains: in other galaxies
- •grains, interstellar: destruction and formation
- •grains, interstellar: size and composition
- •grain size
- •grain size analysis
- •grand potential
- •granulation
- •grating spectrograph
- •gravitation
- •gravitational collapse
- •gravitational constant G
- •gravitational equations
- •gravitational instability
- •gravitational lens
- •gravitational multipole moments
- •gravitational perturbations
- •gravitational potential
- •gravitational radius
- •gravitational redshift
- •gravitational wave
- •graviton
- •gravity
- •gravity anomaly
- •gravity assist
- •grazing incidence optics
- •Great Attractor
- •Great Red Spot
- •great salinity anomaly (GSA)
- •Greenhouse Effect
- •greenhouse gases
- •green line
- •Green’s theorem
- •Greenwich mean (solar) time (GMT)
- •Greenwich sidereal date (GSD)
- •Gregorian
- •Gregorian calendar
- •grism
- •groin
- •gross photosynthetic rate
- •ground level event (GLE)
- •ground stroke
- •groundwater
- •group velocity
- •growing season
- •G star
- •Guiana current
- •guiding center
- •guiding center approximation (GCA)
- •gulf stream
- •guyot
- •gyrofrequency
- •gyroradius
- •Hadley (cell) circulation
- •Hadley cell
- •hadron
- •hail
- •hailstone
- •Hale cycle
- •Hale–Nicholson Polarity Law
- •Halley’s comet
- •halo
- •halocline
- •Hamiltonian
- •Hamilton–Jacobi Theory
- •hard freeze
- •hard radiation
- •Hard X-ray Telescope (HXT)
- •harmonic analysis
- •harmonic model
- •heat capacity
- •Heaviside, Oliver (1850–1925)
- •heavy minerals
- •Hebe
- •Helene
- •heliacal rising
- •helicity
- •heliocentric
- •heliopause
- •helioseismology
- •heliosheath
- •Helios mission
- •heliosphere
- •heliospheric current sheet (HCS)
- •heliospheric stream structure
- •heliospheric termination shock
- •helium
- •helium burning
- •helmet streamer
- •Helmholtz free energy (free energy)
- •Helmholtz theorem
- •Herbig Ae/Be star
- •herringbone burst
- •Hertz (Hz)
- •Hertzsprung–Russell diagram
- •Hesperian
- •Higgs mechanism
- •higher derivative theories
- •highlands, lunar
- •high-pressure and high-temperature experiment
- •HI region
- •Himalia
- •hindcasting (wave)
- •historical climate
- •hoarfrost
- •Holmberg radius
- •homogeneity
- •homologous temperature (
- •homothety
- •homotopy group
- •hopper dredge
- •horizon
- •horizontal branch star
- •horse latitudes
- •horst
- •hot dark matter
- •hot spot
- •hot towers
- •hour angle
- •Hubble diagram
- •Hubble parameter (Hubble constant)
- •Hubble radius
- •Hubble–Reynolds law
- •Hubble sequence
- •Hubble’s law
- •Hubble space telescope (HST)
- •Hubble time
- •humic substance
- •hurricane
- •Huygens principle
- •Hyades
- •hybrid topological defect
- •hydraulic head (piezometric head)
- •hydraulic conductivity (
- •hydraulic depth
- •hydraulic-fracturing method
- •hydraulic gradient (
- •hydraulic head (
- •hydraulic jump
- •hydraulic radius
- •hydraulic routing
- •hydraulic transmissivity
- •hydrodynamic
- •hydrodynamic instability
- •hydrogen
- •hydrogen burning
- •hydrograph
- •hydrographic survey
- •hydrologic equation
- •hydromagnetic
- •hydromagnetics
- •hydromagnetic shock wave
- •hydromagnetic turbulence
- •hydromagnetic wave
- •hydrosphere
- •hydrostatic
- •hydrostatic approximation
- •hydrostatic equation
- •hydrostatic equilibrium
- •hydrostatic pressure
- •Hygiea
- •hygrometer
- •Hyperion
- •hypersonic
- •hysteresis
- •Iapetus
- •Icarus
- •ideal gas
- •ideal gas [equation of state]
- •igneous
- •igneous rocks
- •illuminance (lux)
- •immersed weight
- •impact basin
- •impact crater
- •impact crater ejecta
- •impact creep
- •impact excavation phase
- •impact polarization
- •impact velocities
- •impermeable
- •inclination
- •incompatible element
- •index, geomagnetic activity
- •index of refraction (n)
- •indices, geomagnetic
- •induced Compton scattering
- •induced gravity
- •inelastic collision
- •inelastic scattering of radiation
- •inertia
- •inertial-convective subrange
- •inertial coordinate system
- •inertial frequency
- •inertial instability
- •inertial mass
- •inertial oscillation
- •inertial subrange
- •inferior conjunction
- •inferior mirage
- •infrared
- •infrasound
- •inherent optical property (IOP)
- •inhomogeneous models (of the universe)
- •initial condition
- •initial data
- •initial mass function (IMF)
- •injection boundary
- •inner core
- •inner radiation belt
- •inshore
- •insolation
- •intensity
- •interaction of galaxies
- •intercloud medium
- •intercommutation
- •interface-controlled creep
- •interference fading
- •interglacial
- •interior Schwarzschild space-time
- •intermediate shock
- •intermittency
- •internal energy
- •internal friction angle
- •internal wave
- •International Atomic Time (TAI)
- •international dateline
- •International System of Units (SI)
- •International Temperature Scale (ITS-90)
- •interplanetary dust particles (IDPs)
- •interplanetary magnetic sector
- •interplanetary propagation
- •interplanetary scintillation (IPS)
- •interplanetary scintillation (IPS) observations
- •interplanetary sector structure
- •interplanetary shock wave
- •interplanetary stream structure
- •interplate earthquake
- •interstellar clouds
- •interstellar dust
- •interstellar gas
- •interstellar medium
- •interstellar molecules
- •intertropical convergence zone (ITCZ)
- •intraplate earthquake
- •intrinsic permeability (
- •intrusion
- •invariant
- •inverse Compton effect
- •inverse Compton radiation
- •inverse problems
- •inverse theory
- •inverted barometer effect (inverse barometer response)
- •ion acoustic waves
- •ionization
- •ionization equilibrium
- •ionizing radiation
- •ionogram
- •ionosonde
- •ionosphere
- •ionospheric absorption
- •ionospheric index
- •ionospheric polarization
- •ionospheric propagation mode
- •ionospheric radio propagation path
- •ionospheric refraction
- •ionospheric regions
- •ionospheric sounder
- •ionospheric sounding
- •ionospheric storm
- •ionospheric variability
- •ion torus
- •ion trap
- •IRAF (Image Reduction and Analysis Facility)
- •Iris
- •IRIS (Incorporated Research Institutions for Seismology)
- •iron
- •line
- •iron meteorite
- •irradiance
- •irregular galaxies
- •irregular waves
- •irribarren number
- •irrotational
- •ISC (International Seismological Centre)
- •isentropic coordinate
- •island arc
- •isobar
- •isobaric coordinate
- •isogonic lines
- •isoline
- •isometry
- •isopycnal processes
- •isostacy
- •isotherm
- •isothermal atmosphere
- •isotope
- •isotope delta value (
- •isotope fractionation factor (
- •isotropic
- •isotropic turbulence
- •isotropy
- •Jacobian
- •Jacobi ellipsoid
- •Jansky (Jy)
- •Janus
- •Japan current
- •Jeans escape
- •Jeans instability
- •Jeans length
- •Jerlov water type
- •jet stream
- •jetty
- •joints
- •JONSWAP
- •Joule (J)
- •Joule heating
- •Jovian planet
- •Julian calendar
- •Julian Date Systems Prior to 1972
- •Transformations among JD, MJD, and Gregorian calendar date
- •Julian year
- •Juliet
- •Junge particle distribution
- •Juno
- •Jupiter
- •katabatic wind
- •k-correction
- •Kelvin–Helmholtz instability
- •Kelvin material
- •Kelvin temperature scale
- •Kelvin (Thompson) circulation theorem
- •Keplerian map
- •Kepler shear
- •Kepler’s laws
- •Kepler’s supernova (SN1604, 3C358)
- •Kerr black hole
- •Kerr metric
- •Keulegan–Carpenter Number
- •K-function
- •Kibble mechanism
- •Kibel, I.A.
- •Killing horizon
- •Killing tensor
- •Killing vector
- •K index
- •kinematical invariants
- •kinematics
- •kinematic viscosity
- •kinetic energy
- •kinetic temperature
- •kink
- •Kirchoff’s law
- •Kirkwood gaps
- •knoll
- •knot
- •Knudsen number
- •Kolmogorov scale (Kolmogorov microscale)
- •Kolmogorov spectrum
- •Kp index
- •KREEP
- •Kruskal extension (1960)
- •krypton (Kr)
- •K star
- •Kuiper belt
- •Kuiper belt object, trans-Neptunian object, Edgeworth–Kuiper object
- •Kuroshio
- •lagoon
- •Lagrange points
- •Lagrangian
- •Lagrangian coordinates
- •Lagrangian coordinates
- •Lagrangian representation
- •Lagrangian velocity
- •Laing–Garrington effect
- •Lambertian surface
- •Lambert’s law
- •Lamé constants
- •Landau damping and instability
- •Langmuir circulation
- •Langmuir waves
- •Laplace equation
- •lapse rate
- •Large Magellanic Cloud (LMC)
- •large-scale
- •Larissa
- •Larmor frequency
- •last scattering
- •latent heat
- •latitude
- •lattice preferred orientation (LPO)
- •Laurasia
- •lava
- •law of equal areas
- •law-of-the-wall layer
- •law-of-the-wall scaling
- •laws of black hole physics
- •layering
- •leader spot
- •leap second
- •leap year
- •Leda
- •lee wave
- •left-lateral strike-slip fault
- •Lemaître, Georges
- •Law-of-the-Wall Scaling Relations
- •Lemaître–Tolman cosmological model
- •length of day
- •lens
- •Lense–Thirring precession
- •lepton
- •leveling (survey)
- •Lewis number
- •Lg wave
- •libration
- •Lie derivative
- •lifting condensation level
- •light bridge
- •light cone
- •light-curve
- •light-harvesting complex
- •lightlike current (cosmic string)
- •lightning
- •light pollution
- •light year (l.y.)
- •LIGO (Laser Interferometric Gravitational Observatory)
- •limb
- •limb brightening
- •limb darkening
- •Lindblad resonance
- •linearized gravitation
- •linear momentum
- •linear wave theory
- •line of apsides
- •line of nodes
- •LINER
- •line squall
- •line wing
- •Liouville’s theorem (the equation of continuity)
- •liquefaction
- •LISA (Laser Interferometric Space Antenna)
- •lithophile
- •lithosphere
- •lithostatic pressure
- •little ice age
- •littoral
- •littoral barrier
- •littoral cell
- •littoral current
- •littoral drift
- •littoral drift rose
- •littoral transport
- •lobe
- •lobe dominated quasars
- •lobes, high latitude
- •local acceleration of gravity
- •Local Group
- •local thermodynamic equilibrium (LTE)
- •local topological defect
- •loess
- •Long Duration Event (LDE)
- •longitude, terrestrial
- •longitudinal invariant
- •longitudinal wave
- •long period variables
- •longshore bar
- •longshore current
- •longshore sediment transport
- •long slit spectroscopy
- •Lorentz boost
- •Lorentz factor,
- •Lorentz–Fitzgerald contraction
- •Lorentz force equation
- •Lorentzian metric
- •Lorentz invariance
- •Lorentz transformation
- •Lorentz transformation [implications]
- •Love numbers
- •Love wave
- •lower hybrid waves
- •lower mantle
- •Lowes power spectrum
- •Lowest Useable Frequency (LUF)
- •Low frequency radio emission from planets
- •luminosity class
- •luminosity distance
- •luminosity function of galaxies
- •luminous blue variables
- •Luminous power (lumens)
- •lunar eclipse
- •lunar highlands
- •lunar libration
- •Lunar Maria
- •lunar mascons
- •lunar meteorites
- •Lunar Prospector
- •Lunar Rille
- •Lyapunov exponent
- •Lyman limit
- •Lyman series
- •Lysithea
- •Mach, Ernst (1838–1916)
- •Mach’s principle
- •Maclaurin Spheroid
- •macroscopic description (cosmic string)
- •Madden–Julian oscillation (MJO)
- •Magellanic Clouds
- •Magellan Mission
- •magma
- •magma chamber
- •magmatic water
- •magnetic anomaly
- •magnetic bay
- •magnetic carpet
- •magnetic cloud
- •magnetic crochet
- •magnetic declination
- •magnetic diffusivity
- •magnetic dipole
- •magnetic helicity
- •magnetic inclination
- •magnetic latitude
- •magnetic local time (MLT)
- •magnetic longitude
- •magnetic mirror
- •magnetic pole
- •magnetic pressure
- •magnetic reconnection
- •magnetic regime (cosmic string)
- •magnetic reversal
- •magnetic Reynolds number
- •magnetic secular variation
- •magnetic shear
- •magnetic tension
- •magnetism
- •magnetoacoustic wave
- •magnetogram
- •magnetohydrodynamics (MHD)
- •magnetometer
- •magnetopause
- •magnetosonic wave
- •magnetosphere
- •magnetospheric substorms
- •magnetostratigraphy
- •magnetostrophic
- •magnetotail
- •magnitude
- •Maia
- •main sequence star
- •major axis
- •Manning’s equation
- •manometer
- •mantle
- •mantle convection
- •mantle transition zone
- •March 5th event
- •mare
- •Marianas Trench
- •marine snow
- •Markarian galaxies
- •Mars
- •Mars Climate Orbiter (MCO)
- •Mars Global Surveyor (MGS)
- •Mars Microphone
- •Mars Microprobe
- •Mars Polar Lander (MPL)
- •Martian geophysical epochs
- •Martian meteorites
- •mascon
- •maser
- •mass-defect
- •mass extinctions
- •massive ghost
- •mass-luminosity relation
- •mass transfer
- •mass wasting
- •matter density perturbations
- •Maunder Minimum
- •maximal extension of a space-time
- •maximally rotating black hole
- •maximally symmetric space
- •Maximum Observable Frequency (MOF)
- •Maxwell–Boltzmann distribution
- •Maxwell material
- •mean anomaly, M
- •mean celestial equator
- •mean celestial pole
- •mean cosine of scattering angle
- •mean diameter, mean grain size
- •mean free path
- •mean motion
- •mean motion (short-period) resonances
- •mean sea level
- •mean solar day
- •mean solar time
- •mean water level
- •mechanics
- •mechanics, Newtonian
- •median diameter, median grain size
- •Meiyu front
- •membrane paradigm
- •Mercalli intensity scale
- •Mercury
- •merger
- •merging, magnetic
- •Merian’s equation
- •meridian
- •meridional
- •meromixis
- •Merope
- •mesogranulation
- •meson
- •mesoscale
- •mesosphere
- •mesotrophic water
- •Messier
- •metallic hydrogen
- •metallicity
- •metamorphism
- •meteor
- •meteorite
- •meteorite parent body
- •meteoroid
- •meteor shower
- •methane
- •Metis
- •metric
- •metricity of covariant derivative
- •metric radio burst
- •MHD condition
- •MHD simulation
- •Michelson Doppler Imager/Solar Oscillations Investigation (MDI/SOI)
- •Michelson–Morley experiment
- •Miche–Rundgren theory
- •micrometeorite
- •microstructure
- •microwave background radiation
- •microwave burst
- •Mie scattering
- •Mie size parameter
- •migration (seismic)
- •Milankovich cycle
- •Miles–Phillips–Hasselmann theory
- •millibar
- •Mimas
- •minimal coupling
- •minisuperspace
- •minor axis
- •Mira
- •mirage
- •Miranda
- •mirror instability
- •mixed layer
- •mixing length
- •mixing ratio
- •Mixmaster universe
- •M magnitude
- •model atmosphere
- •mode water
- •modulation of galactic cosmic rays
- •Mohr’s circle
- •moist lapse rate
- •molar gas constant (
- •molecular cloud
- •molecular torus
- •moment
- •moment of inertia
- •Monin–Obukhov length
- •monopole
- •monopole annihilation
- •monopole excess problem
- •monsoon
- •monsoon climate
- •month
- •moody diagram
- •moon
- •moonbow
- •moraines
- •Moreton wave
- •morning cloud (Mars)
- •morphodynamics
- •morphology-density relationship
- •mountain climate
- •moving magnetic features
- •M star
- •MUF fading
- •multicell storm
- •multipath fading
- •multiple ring basins
- •multiple shock
- •mushy zone
- •nadir
- •nadir angle
- •Naiad
- •naked singularity
- •nakhlite
- •nanotesla (nT)
- •narrow emission line galaxies
- •Narrow Line Region (NLR)
- •Nasmyth universal spectrum
- •natural line broadening
- •natural remanent magnetism
- •nautical mile
- •nautical twilight
- •neap tide
- •NEAR
- •nearly diurnal free wobble
- •nebular hypothesis
- •nebular lines
- •neon
- •neon burning
- •Neptune
- •Nereid
- •net photosynthetic rate
- •network
- •Neupert effect
- •neutral equilibrium
- •neutral point
- •neutral stability
- •neutrino
- •neutrino annihilation
- •neutrino viscosity
- •neutron albedo
- •neutron star
- •neutron star kicks
- •Newman–Penrose formalism
- •New Millennium Program (NMP)
- •Newtonian
- •Newtonian cosmology
- •Newtonian gravitational constant
- •Newtonian gravity
- •Newtonian invariance
- •Newtonian relativity
- •Newtonian simultaneity
- •Newton (N)
- •Newton’s laws of motion 1.
- •Nielsen–Olesen vortex
- •nimbus
- •nitrogen
- •Noachian
- •nocturnal
- •nodal month
- •node
- •nonderivative absorption
- •nonminimal coupling
- •nonsimultaneous Big Bang
- •non-thermal line broadening
- •non-thermal radiation
- •non-thermal spectral energy distribution
- •non-topological soliton
- •nonuniqueness
- •norite
- •normal fault
- •normal incidence frame
- •normal incidence optics
- •normal modes
- •North Atlantic current
- •North Atlantic Oscillation (NAO)
- •North Equatorial current
- •Northern Plains (Mars)
- •nova
- •nuclear fusion
- •nuclear reactions
- •nuclear time scale
- •nuclear winter
- •nucleation
- •nucleus
- •nucleus (of a comet)
- •null vector
- •numerical cosmology (computational cosmology)
- •numerical model
- •numerical relativity
- •Nusselt number
- •nutation
- •obduction
- •Oberon
- •objective grating
- •objective prism
- •oblateness
- •oblique ionogram
- •oblique-slip fault
- •obliquity
- •obliquity factor
- •Occam’s razor
- •occluded front
- •occultation
- •ocean
- •ocean color
- •ocean color sensor
- •oceanic optics
- •Ockham’s razor
- •offshore
- •Olbers paradox
- •oligotrophic water
- •Olympus Mons
- •omega bands
- •One-form, 1-form
- •Oort cloud
- •open cluster
- •open magnetosphere
- •open ocean
- •open universe
- •operational MUF
- •Ophelia
- •ophiolites
- •opposition
- •optical closure in measurement
- •optical closure in models
- •optical closure in scale
- •optical constants
- •optical density
- •optical depth
- •optical distance
- •optical double star
- •optical oceanography
- •Optimum Working Frequency (OWF)
- •orbital elements
- •orogeny
- •orographic cloud
- •Orowan’s equation
- •orthogonal
- •Osborn–Cox method
- •Osborn model
- •oscillating universe
- •O star
- •outer core
- •outfall
- •outgoing longwave radiation (OLR)
- •oval equation
- •overtopping
- •overturning scale
- •overwash
- •OVV quasar
- •oxygen
- •Ozmidov scale
- •ozone
- •ozone layer
- •ozonosphere
- •package effect
- •paleoclimate
- •palimpsest
- •Pallas
- •pancake distribution
- •pancake dome
- •Pandora
- •Pangaea
- •Papapetrou spacetimes (1953)
- •paraboloid
- •parallax
- •Parker limit
- •parsec
- •partial derivative
- •partial pressure
- •particle acceleration
- •particle horizon
- •pascal (Pa)
- •Paschen series
- •Pasiphae
- •passive continental margin
- •passive earth force
- •patchiness and intermittence
- •patera
- •pathline
- •Peclet number
- •peculiar motion
- •peculiar velocity
- •peeling property
- •penetrative convection
- •Penman equation (or combination equation)
- •Penrose diagram
- •Penrose process
- •penumbra
- •percolation
- •perfect gas
- •periapsis
- •periastron
- •perihelion
- •perihelion shift
- •period
- •period-luminosity relation
- •permafrost
- •permeability
- •persistent current
- •perturbation theory
- •perturbative solution
- •Peru current
- •Petchek reconnection
- •Petrov types
- •Petzold data
- •Pfund series
- •phase
- •phase angle
- •phase frequency threshold
- •phase function
- •phase space
- •phase speed
- •phase transition
- •phase velocity
- •pheophytin
- •phi unit
- •Phobos
- •Phoebe
- •photodissociation region
- •photoelectrons
- •photoinhibition
- •photoionization
- •photometric binary
- •photometry
- •photon
- •photosphere
- •photosynthesis
- •photosynthetically available radiation
- •photosynthetic capacity
- •photosynthetic pigment
- •phytoplankton
- •piezometer
- •piezometric head
- •Pileus cloud
- •pitch angle
- •pitch angle diffusion
- •Pitot tube
- •plage
- •plagioclase (triclinic feldspar)
- •Planck constant
- •Planck length
- •Planck mass
- •Planck time
- •planet
- •planetary boundary layer
- •planetary circulation
- •planetary magnetosphere
- •planetary nebula
- •planetary radio astronomy
- •planetary rotation periods
- •planetesimal
- •plankton
- •plankton bloom
- •plasma
- •plasma frequency
- •plasma mantle
- •plasma sheet boundary layer (PSBL)
- •plasmasphere
- •plasma stress tensor
- •plastic anisotropy
- •plastic (permanent) deformation
- •plateau material
- •plate tectonics
- •platonic year
- •Pleiades
- •Pleione
- •Pluto
- •pluton
- •plutonic
- •pocket beach
- •point object
- •point spread function (PSF)
- •polar cap
- •polar cap absorption (PCA)
- •polar cap arc (or sunward arc)
- •polar cap (Mars)
- •polar cap precipitation
- •polar crown
- •polar cusp
- •polar dunes
- •polar glow
- •polar hood (Mars)
- •Polaris
- •polarization
- •polarization brightness
- •polarization fading
- •polarization state
- •polar motion
- •polar plume
- •polar wander
- •polar wind
- •pole-on magnetosphere
- •poles of Mars
- •Pole star
- •Pollux
- •poloidal/toroidal decomposition
- •polycyclic aromatic hydrocarbons (PAHs)
- •polynya
- •polytropic process
- •poorly graded sediment
- •Population I
- •Population II
- •poroelastic medium
- •Portia
- •position vector
- •positron
- •POSS
- •postglacial rebound
- •post-seismic relaxation
- •potassium-argon age
- •potential
- •potential density
- •potential energy
- •potential height
- •potential instability
- •potential temperature
- •potential theory
- •potential vorticity
- •potentiometric surface (or piezometric surface)
- •power
- •p process
- •prairie
- •Prandtl number
- •Pratt compensation
- •Pratt isostasy
- •precession
- •precession of the equinoxes
- •precipitation
- •precursor
- •pressure
- •pressure altitude
- •pressure anisotropy
- •pressure coordinate
- •pressure head (
- •primary production
- •primary productivity
- •primary rainbow
- •prime focus
- •primordial black holes
- •principal null directions
- •principal spinors
- •principle of equivalence
- •probable maximum hurricane
- •progressive vector diagram
- •progressive wave
- •Prometheus
- •prominence
- •proper time
- •proplyd
- •Proteus
- •proton
- •protostar
- •protosun
- •protuberance
- •Proxima Centauri
- •psychrometric constant
- •psychrometer
- •pseudovector
- •pseudobreakup
- •Puck
- •pulsar
- •pulsar velocities
- •pycnocline
- •pycnostad
- •pyconuclear reaction
- •pyroclastic
- •pyroxene
- •Q factor
- •quadrupole formula
- •quanta meter
- •quantization of redshift
- •quantum gravity
- •quantum yield
- •quark
- •quasar
- •quasar-galaxy association
- •quasi-biennial oscillation
- •quasi-hydrostatic approximation
- •quasi-neutral equilibrium
- •quasi-separatrix layer
- •quasi-single-scattering approximation
- •quasi stellar object
- •quasi-viscous force
- •quay
- •quenching
- •quicksand
- •quiet sun
- •radar
- •radian
- •radiance
- •radiation
- •radiation belts
- •radiation cooling
- •radiation gauge
- •radiation pressure
- •radiation tide
- •radiation zone
- •radiative-convective equilibrium
- •radiative transfer equation
- •radio absorption
- •radioactive
- •radioactive decay
- •radio burst
- •radio core
- •radio emission: types I-IV
- •Radio Frequency Bands
- •radio frequency interference (RFI)
- •radio frequency spectrum
- •radio galaxy
- •radioisotopes
- •radio lobe
- •radiometer
- •radiometric dating
- •radiometry
- •radio stars
- •radius of deformation
- •radon
- •rain
- •rainbow
- •Raman scattering
- •random waves
- •Rankine–Hugoniot relations
- •rare earth elements
- •Raychaudhuri equation
- •Rayleigh distribution
- •Rayleigh–Jeans approximation
- •Rayleigh number
- •Rayleigh scattering
- •Rayleigh wave
- •ray parameter
- •ray tracing
- •ray tracing: wave packet approximation
- •R Coronae Borealis stars
- •real singularity
- •recombination (in atomic and molecular physics)
- •recombination line
- •reconnection
- •recurrent novae
- •red giant
- •red line
- •redshift
- •Red Spot
- •reduced gravity
- •reduced gravity model
- •reef
- •reference frame
- •refracting telescope
- •refraction
- •refractive index
- •refractory
- •region of anomalous seismic intensity
- •Regolith
- •Regulus
- •relative depth
- •relative humidity
- •relativistic jets
- •relativistic time delay
- •relativity
- •relaxation time
- •residual circulation
- •resolution
- •resonance
- •resonance scattering
- •resonant absorption
- •resonant damping and instability
- •resonant layer
- •rest mass
- •retrograde motion
- •retrograde orbit
- •retrograde rotation
- •return current
- •return stroke
- •reverse fault
- •reversible process
- •revetment
- •Reynolds decomposition
- •Reynolds number (
- •Reynolds stress
- •Rhea
- •rheology
- •Ricci tensor
- •Richard’s equation
- •Richardson, Lewis F.
- •Richardson number
- •Richter magnitude scale
- •ridge push
- •ridge slide (ridge push)
- •Riemann-Christoffel tensor
- •Riemann tensor
- •Rigel
- •right ascension
- •right-hand coordinate system
- •right-lateral strike-slip fault
- •rigidity
- •rigid lid approximation
- •rille
- •Rindler observer
- •ring
- •ring current
- •ring galaxy
- •riometer
- •rip current
- •riprap
- •Robertson–Walker cosmological models
- •Robinson–Trautman space-time (1962)
- •Roche limit
- •Roche lobe
- •Rocket effect (string loop)
- •Rosalind
- •Rossby number
- •Rossby radius
- •Rossby radius of deformation
- •Rossby wave
- •rotation
- •rotational discontinuity
- •rotation curve
- •rotation of the universe
- •r process
- •RS Canum Venaticorum stars
- •runoff channels
- •runup
- •RV Tauri stars
- •Sagittarius A*
- •salinity
- •salinity inversion
- •saltation
- •salt wedge
- •sand budget
- •sand bypassing
- •sand spit
- •sand trap
- •Sargasso Sea
- •saros
- •satellite altimetry
- •Satellite Laser Ranging (SLR)
- •saturation
- •saturation adiabat
- •saturation adiabatic lapse rate
- •saturation vapor pressure
- •Saturn
- •scalar
- •scale factor
- •scaling solution (cosmic string and cosmic texture)
- •scarps
- •scatterance
- •scattered disk, scattered disk object
- •scatter-free shock acceleration
- •scattering albedo
- •scattering angle
- •scattering cross-section
- •Schmidt camera
- •Schwarzschild black hole
- •Schwarzschild metric (Schwarzschild solution)
- •Schwarzschild solution
- •Schwarzschild spacetime
- •scintillation
- •scour
- •sea level
- •seamount
- •seasonal thermocline
- •sea stack
- •seawall
- •SeaWiFS
- •Secchi depth
- •Secchi disk
- •second
- •secondary craters
- •secondary rainbow
- •second fundamental form
- •sector boundary
- •sector structure
- •secular (long-period) resonances
- •sedimentary
- •sedimentary basin
- •sediment budget
- •sediment load
- •sediment sink
- •sediment source
- •sediment transport
- •seeing
- •seiche
- •seismic coupling factor
- •seismic gap
- •seismic intensity
- •seismicity
- •seismic moment
- •seismic parameter
- •seismic wave
- •seismogram
- •seismograph
- •seismology
- •seismometer
- •seismotectonics
- •selective heating
- •self-generated turbulence
- •semiclassical gravity
- •semimajor axis
- •separator
- •separatrix
- •sequence stratigraphy
- •Sérsic–Pastoriza (1965) galaxies
- •seston
- •setdown
- •settling speed (velocity)
- •settling tube
- •setup
- •Seyfert-1 galaxies
- •Seyfert galaxies
- •sferic
- •shallow water
- •shallow water wave
- •shatter cone
- •shear
- •shear modulus
- •shear probe
- •shear stress
- •shear velocity
- •shear wave
- •shear wave splitting
- •sheet pile
- •shelf wave
- •shepherding moon
- •shepherd satellite
- •shergottite
- •shields diagram
- •shield volcano
- •shingle
- •shoal
- •shoaling
- •shock normal
- •shock spike
- •shock wave
- •shoreline
- •short wave fadeout (SWF)
- •sidereal day
- •sidereal month
- •sidereal time
- •sidereal year
- •siderophile
- •sieve analysis
- •Sievert
- •signature
- •sign convention
- •silent earthquake
- •silent universe
- •silicon burning
- •silt
- •Simon moments (1984)
- •simple harmonic motion
- •simple harmonic wave
- •simple pendulum
- •simultaneity, in Newtonian mechanics
- •single scattering albedo
- •single scattering phase function
- •singularities
- •singularity theorems
- •sinistral fault
- •Sinope
- •sinusoidal wave
- •Sirius
- •SI (Systeme International)
- •skewness
- •skip fading
- •skip zone
- •Skylab
- •slab model
- •slab penetration
- •slip system
- •slough
- •slow earthquake
- •slow magnetohydrodynamic shock
- •slow shock wave
- •slow solar wind
- •small amplitude wave
- •Small Magellanic Cloud (SMC,NGC 292)
- •small-scale turbulence
- •SNC meteorites
- •snow
- •soft gamma repeaters
- •Soft X-ray Telescope (SXT)
- •solar abundance
- •solar activity
- •Solar and Heliospheric Observatory (SOHO)
- •solar atmosphere
- •solar constant
- •solar cycle
- •solar day
- •solar disk
- •solar dynamo
- •solar eclipse
- •solar electromagnetic radiation
- •solar granulation
- •solar limb
- •solar magnetic sectors
- •solar maximum
- •solar maximum mission (SMM)
- •solar minimum
- •solar nebula
- •solar neutrinos
- •solar P-angle
- •solar quiet current system
- •solar radiation
- •solar rotation
- •solar spectral irradiance
- •solar system
- •solar system formation
- •solar wind
- •solar year
- •solar zenith angle
- •solid Earth tides
- •solitary wave
- •soliton
- •solstice
- •sound wave
- •source functions
- •source surface
- •South Atlantic Anomaly
- •South Equatorial current
- •southern oscillation
- •Southern Oscillation Index (SOI)
- •spacetime
- •space weather
- •spallation
- •special relativity
- •spectral
- •spectral energy distribution
- •spectral energy distribution of active galactic nuclei
- •spectral gap
- •spectral line
- •spectral type
- •spectrograph
- •spectrometer
- •spectrophotometry
- •spectropolarimetry
- •spectroradiometer
- •spectroscopic binary
- •spectroscopy
- •spectrum
- •spectrum binary
- •speed of light
- •spherical harmonic analysis
- •spherical pendulum
- •Spica
- •spicule
- •spinel
- •spinor
- •spin up
- •spiral arm
- •spiral galaxy
- •spontaneous symmetry breaking
- •sporadic E
- •spread E
- •spread F
- •spring
- •spring tide
- •sprites
- •squall
- •s (slow) process
- •S stars
- •stability frequency
- •stability of the water column
- •stability ratio
- •stable auroral red (SAR) arcs
- •stable causality
- •stable equilibrium
- •standard atmosphere
- •standard candle
- •standard pressure
- •standard project hurricane
- •standard temperature and pressure
- •standard time
- •standing shock
- •standing wave
- •Stanton number (
- •starburst galaxy
- •Stardust
- •star formation
- •star formation rate (SFR)
- •Stark effect
- •star spots
- •statcoulomb
- •state parameter
- •steepness (wave)
- •stellar activity
- •stellar evolution
- •stellar population
- •stellar winds
- •stepped leader
- •steric height
- •stick-slip
- •still water level
- •stishovite
- •stochastic acceleration
- •Stokes parameters
- •Stokes parameters
- •Stokes polarimetry
- •Stokes wave theory
- •Störmer orbits
- •Störmer theory
- •Störmer unit
- •storm sudden commencement (SSC)
- •storm surge
- •storm track
- •strain
- •strain hardening
- •strain partitioning
- •strain softening
- •stratosphere
- •stratovolcano
- •stratus
- •streakline
- •streamer belt
- •stream function wave theory
- •streamline
- •stress
- •stress drop
- •stress energy tensor
- •striations, auroral
- •string model
- •string network
- •stromatolite
- •strong anthropic principle
- •strong scattering limit
- •Strouhal number (
- •structure toe
- •St. Venant Equations
- •subduction
- •subduction zone
- •subdwarf
- •subgiant
- •subgrain size
- •submarine canyon
- •subsidence
- •subsolar point
- •subsonic string model
- •substorm injection
- •substorm phases
- •substorm triggering
- •substorm wedge
- •subtropical high-pressure belt
- •sudden frequency deviation (SFD)
- •sudden ionospheric disturbance (SID)
- •sudden phase anomaly (SPA)
- •sudden stratospheric warming
- •sulfur dioxide
- •summation convention
- •summer solstice
- •sunlit aurora
- •sunspot
- •sunspot cycle
- •sunspot number
- •sunspot number (daily)
- •sunspot number (smoothed)
- •sunward arc
- •supercell storm
- •super cloud cluster
- •supercluster
- •superconducting string
- •supercooling
- •supergeostrophic wind
- •supergiant
- •supergradient wind
- •supergranulation
- •superior conjunction
- •superior mirage
- •superluminal source
- •supermassive black hole
- •supernova
- •supernovae, 1987A
- •supernovae, 1991bg
- •supernovae, 1993J
- •supernovae, accretion induced collapse (AIC)
- •supernovae, core collapse mechanism
- •supernovae, delayed neutrino mechanism
- •supernovae, distance indicators
- •supernovae, expanding photosphere method
- •supernovae, fallback
- •supernovae, gravitational waves
- •supernovae, light curves
- •supernovae, neutrino detectors
- •supernovae, neutrino-driven wind mechanism
- •supernovae, neutrinos
- •supernovae, prompt shock mechanism
- •supernovae, spectra
- •supernovae, thermonuclear explosions
- •supernovae, Type Ia
- •supernovae, Type Ib/Ic
- •supernovae, Type II
- •supernovae, white dwarf accretion
- •supernova rates
- •supernova remnant (SNR)
- •Supernova Rates
- •superposition principle
- •supersonic
- •supersonic string model
- •superspace
- •surface boundary layer
- •surface gravity
- •surface tension (
- •surface waves
- •surf zone
- •surge
- •suspended load
- •suspension
- •suture
- •swash zone
- •swell
- •symbiotic star
- •symmetric instability
- •symmetry
- •synchronous orbit
- •synchronous rotation
- •synchrotron radiation
- •synchrotron self-Compton mechanism
- •synodical month
- •synodic month
- •synodic period
- •synoptic scale
- •synthetic aperture radar
- •syzygy
- •tachyon
- •tail current
- •Talwani’s method
- •tangential discontinuity
- •tangential geostrophy
- •Taygeta
- •Taylor instability
- •Taylor number
- •Taylor’s hypothesis
- •Taylor state
- •tectonics
- •tectosphere
- •Telesto
- •temperature
- •temperature inversion
- •temperature variance dissipation rate
- •tension (cosmic string)
- •tension head (ψ)
- •tensor
- •terminal velocity
- •terminator
- •Terrestrial Coordinate Time (TCG)
- •Terrestrial Dynamical Time (TDT)
- •terrestrial planets
- •Terrestrial Time (TT)
- •tesla
- •Tethys
- •Tethys Ocean
- •texture
- •Thalassa
- •Tharsis Province
- •Thebe
- •thermal anisotropy
- •thermal bar
- •thermal boundary layer
- •thermal bremsstrahlung
- •thermal conductivity
- •thermal diffusive sublayer
- •thermal diffusivity
- •thermal Doppler line broadening
- •thermal expansivity
- •thermal wind relationship
- •thermobaric effect
- •thermobaricity
- •thermocline
- •thermohaline circulation
- •thermosphere
- •Theta aurora
- •thick-target
- •thin-target
- •30 Doradus
- •Thompson circulation theorem
- •Thomson scattering
- •Thorpe displacement
- •Thorpe scale
- •thrust fault
- •Tibetan Plateau
- •tidal bore
- •tidal currents
- •tidal delta
- •tidal energy dissipation
- •tidal forces
- •tidal formation of solar system
- •tidal friction
- •tidal heating
- •tidal inlet
- •tidal period
- •tidal prism
- •tidal radius
- •tidal stripping
- •tidal tail
- •tide
- •tide range
- •tilt angle
- •time dilatation
- •time dilation
- •time-distance helioseismology
- •time in semiclassical gravity
- •time zone
- •Titan
- •Titania
- •Tolman model
- •tombolo
- •Tomimatsu–Sato metrics (1973)
- •Toomre’s stability parameter
- •TOPEX/POSEIDON
- •Top hat detector
- •topocentric system
- •topographic wave
- •topography
- •topological defect
- •topology of space
- •tornado
- •torque
- •torsion
- •torsional oscillation
- •total electron content (TEC)
- •total radiance mean cosine
- •towards polarity
- •tracers
- •traction
- •transcurrent (transform) plate boundary
- •transform boundary
- •transform fault
- •transform push
- •transient shock
- •transitional depth
- •transition region
- •translatory wave
- •transmissivity (
- •transonic string model
- •transparency
- •transpiration
- •transverse Doppler shift
- •transverse wave
- •trapped surface
- •traveling ionospheric disturbance (TID)
- •travel time curve
- •trench
- •trench excavation
- •triggered star formation
- •triple junction
- •triple point
- •tripton
- •tritium
- •trochoidal wave theory
- •Tritium Unit
- •Triton
- •Trojans
- •Trojans asteroids
- •tropical cyclone
- •tropical instability waves
- •tropical month
- •tropical storm
- •tropical year
- •Tropic of Cancer
- •Tropic of Capricorn
- •tropopause
- •troposphere
- •trough
- •true anomaly
- •true celestial equator
- •true celestial pole
- •true north
- •true polar wander
- •tsunami
- •tsunami earthquake
- •T Tauri object
- •T Tauri star
- •turbidity
- •turbulence
- •turbulence cascade
- •turbulent cascade
- •turbulent kinetic energy
- •twilight
- •twin paradox
- •twistor
- •2-form
- •two-stream approximation
- •two-stream instability
- •type I radio burst
- •type II radio burst
- •type III radio burst
- •type IV radio burst
- •typhoon
- •ultrasound
- •ultraviolet
- •Ulysses Mission
- •umbra
- •Umbriel
- •unconformities
- •undertow
- •unipolar magnetic region
- •unit weight
- •universal gravitation, Newton law of
- •Universal Time (UT or UT1)
- •universe
- •unstable equilibrium
- •upper mantle
- •upper neutral atmospheric regions
- •Uranus
- •Urca process
- •Urey ratio
- •V471 Tauri stars
- •vacuum
- •vacuum manifold
- •vacuum polarization
- •Väisälä frequency
- •Valles Marineris
- •valley networks
- •Van Allen Belts
- •VAN method
- •vapor
- •vapor concentration
- •vapor pressure
- •variable star
- •variable stars [cataclysmic variables]
- •variable stars [geometric variables]
- •variable stars [peculiar variables]
- •variable stars [pulsating variables]
- •variational principle
- •Vasyliunas theorem
- •vector
- •vector cross product
- •vector magnetograph
- •velocity
- •velocity curve
- •velocity dispersion
- •velocity distribution
- •velocity strengthening
- •velocity weakening
- •ventifacts
- •ventilated thermocline
- •Venturi tube
- •Venus
- •Venusian Tessera
- •vernal equinox
- •Very Long Baseline Interferometry (VLBI)
- •Vesta
- •virga
- •Virgo
- •Virgo constellation
- •Virgo Supercluster
- •virtual geomagnetic pole
- •viscoelastic material
- •viscosity
- •viscosity
- •viscous-like force
- •viscous shear stress
- •viscous sublayer
- •visible light
- •visible wavelengths
- •visual binary system
- •Vlasov equation
- •voids
- •Voigt body
- •volatile
- •volcanic front
- •volcanic line
- •volcanism
- •volcanos
- •volume scattering function (VSF)
- •volumetric water content
- •von Mises criterion
- •vortex defect of the vacuum
- •vortex street
- •vorticity
- •vorton
- •Wadati–Benioff zones (Benioff zones)
- •wake
- •wake (cosmic string)
- •Walker circulation
- •wall terracing
- •warm front
- •warping (of the neutral sheet or plasma sheet)
- •wash load
- •water
- •water mass
- •waterspout
- •water table
- •watt (W)
- •wave
- •wave-affected surface layer (WASL)
- •wave crest
- •wavefront
- •wave-function of the universe
- •wavelength
- •wave number
- •wave-particle interactions
- •wave rose
- •wave trough
- •wave vector
- •weak anthropic principle
- •weak interaction
- •weakly asymptotically simple space-time
- •weather
- •weather forecast
- •weight
- •weir
- •well-graded
- •Wesselink method
- •westerlies
- •western boundary currents
- •westward drift
- •wetness (or degree of saturation) (S)
- •wetted perimeter
- •Weyl space-times (1917)
- •Weyl tensor
- •whimper
- •whistlers
- •whistler waves
- •white dwarf
- •white hole
- •white light
- •Wien’s displacement law
- •Wien distribution law
- •Wien’s law
- •wiggle (cosmic string)
- •Wilson cycle
- •wind
- •wind chill factor
- •wind-driven circulation
- •wind evaporation-sea surface temperature feedback
- •winding number
- •wind rose
- •wind scale
- •wind shear
- •winter anomaly
- •winter solstice
- •Witten conducting string
- •wolf number
- •work
- •worldsheet charge
- •worldsheet geometry
- •wormhole
- •wrinkle ridges
- •W Ursa Majoris (W UMa)
- •WWSSN (World-Wide Standardized Seismograph Network)
- •xenoliths
- •xenon
- •X-ray source
- •yardangs
- •year
- •Yoshida jet
- •Yukawa coupling
- •Zeeman effect
- •Zeldovich process
- •zenith
- •zenith angle
- •zero mode
- •zodiac
- •zodiacal light
- •zonal
- •zooplankton
- •Zulu Time
- •Zwicky compact galaxies

cosmic microwave background
θ < θ0 will escape the trap, i.e., they thermalize in the ambient plasma before they bounce.
corotating interaction region |
See helio- |
spheric stream structure. |
|
correlation length In phase transitions, topological defects may arise when growing spatial domains with different orientations (phases) of the correlated field fail to match smoothly. Hence, fluctuations in the phase of the field (determined by local physics only) will be uncorrelated on scales larger than a given correlation length ξ, whose details depend on the transition taking place.
The tendency of the field configuration after the transition will be to homogenize, and thus ξ will grow in time. Causality imposes an upper limit, as information cannot propagate faster than light. Hence, in cosmology the correlation length must be smaller than the distance signals can have traveled since the Big Bang, which for both radiationand matter-dominated eras im-
plies ξ |
< |
t, with t the cosmic time. |
The correlation length is of utmost importance for the subsequent evolution of a cosmic defect network. In fact, the initial length scale of the network will be determined by the probability of defect formation out of the coalescence of different domains.
coseismic deformation Displacement such as uplift and subsidence that occurs during an earthquake. The term is used particularly to describe static displacement and not vibration associated with seismic wave propagation. Depending on the means of measurement, the term may represent displacements that occur within a fraction of a second to a period of several years.
cosine collector A radiant energy detector whose effective light collection area is proportional to the cosine of the angle between the incident light and the normal to the detector surface; used to measure plane irradiances.
cosmic abundance The relative abundance of elements in the universe. Hydrogen provides approximately 75% of the mass density of the universe. 4H e provides about 24%. Lithium, beryllium and boron are each at the 10−12 to
10−10 level. These elements are thought to have been produced in the Big Bang.
Heavier elements were produced in stars or supernovae. Carbon, nitrogen, oxygen, and neon are present at parts greater than 10−4. Silicon and iron are abundant at the 10−4 level. Elements with atomic number exceeding approximately 30 are present at the 10−10 to 10−11 level. There is strong “odd-even” effect; even atomic numbers (numbers of protons) or even numbers of neutrons make the isotope much more abundant than nearby isotopes.
cosmic censorship The conjecture put forward by R. Penrose that the formation of naked singularities (singularities visible from infinitely far away) is evaded in nature because singularities in space-time are always surrounded by an event horizon which prevents them from being observed and from influencing the outside world. In this simple formulation the hypothesis was proven false by counterexamples, i.e., models of spacetime whose metrics obey Einstein’s equations, but in which naked singularities exist. According to proponents of cosmic censorship, these examples are not generic. Some of the spacetimes in question are highly symmetric or require tuning of parameters. In others, the gravitational fields in the neighborhood of those singularities are, in a well-defined mathematical sense, too weak (i.e., produce too weak tidal forces) to be considered genuinely singular. There is as yet no well-formulated statement of cosmic censorship or a proof of its holding in general relativity.
cosmic microwave background Single component of cosmic origin that dominates the electromagnetic background at wavelengths in the millimeters to centimeters range. It was serendipitously discovered by Penzias and Wilson in 1965. The cosmic microwave background radiation has the spectrum of a black body at temperature To = 2.728 ± 0.002 K. It can be detected in any direction of the sky. Its high degree of isotropy is an observational evidence that on the largest scale, the universe is homogeneous and isotropic.
The Planckian spectrum of the cosmic microwave background is a strong vindication of the Big Bang picture. Since the universe at
© 2001 by CRC Press LLC

cosmic microwave background, dipole component
present is transparent to radiation (radiogalaxies at redshifts z < 1 are observed at microwave frequencies), a thermal spectrum could not have been produced recently, i.e., at redshifts smaller than unity as would be required by the steady state cosmological model. In the Big Bang model, the effect of the expansion is to decrease the cosmic microwave background temperature, so in the past the universe was hotter. At redshift z, the temperature would be T = To(1+z). At z ≥ 1000 matter and radiation would have achieved thermal equilibrium. In the 1940s, Gamow, Alpher, Herman, and Follin predicted that, as the universe expands and temperature drops, the interactions that kept matter and radiation in thermal equilibrium cease to exist. The radiation that would then propagate freely is the one observed today.
On scales up to a few tens of Mpc, the universe is not homogeneous. The presence of inhomogeneities induces temperature anisotropies on the background radiation. Irregularities in the matter distribution at the moment of recombination, our peculiar motions with respect to the Hubble flow, the effect of hot plasma on clusters of galaxies (see Sunyaev– Zel’dovich effect), and several other contributions, induced anisotropies at the level of one part in 103 (dipole) and at 105 on smaller angular scales. These effects convert the CBR into an excellent probe of the history of structure formation (galaxies, clusters of galaxies) in the evolving universe. See cosmic microwave back- ground, dipole; quadrupole; temperature fluctuations; spectral distortions.
cosmic microwave background, dipole component Dipole variation in the thermodynamic temperature as a function of direction. It is the largest anisotropy present in the cosmic microwave background radiation. The motion of an observer with velocity v with respect to a reference frame where a radiation field (of temperature To) is isotropic produces a Doppler-shifted temperature T (θ) = To(1 +
(v/c)2)1/2/(1−(v/c) cos(θ)) where θ is the angle between the direction of observation and the direction of motion, and c is the speed of light. Immediately after the discovery of the cosmic microwave background, the search started for the Doppler anisotropy described above, and
the first results were obtained at the end of the 1960s. The best-fit of the dipole amplitude is 3.358 ± 0.023 mK in the direction (l, b) = (264◦.31 ± 0◦.16, 48◦.05 ± 0.09) in galactic coordinates. The current understanding is that the largest contribution to the dipole anisotropy comes from the motion of the Earth. All other contributions are negligible. Under this assumption, the data quoted above corresponds to a sun velocity, with respect to the cosmic microwave background, of v = 369.0 ± 2.5 km/s towards the constellation Leo, and the velocity of the local group is vLG = 627 ± 22 km/s in the direction (l, b) = (276o ± 3, 30o ± 2). See peculiar motion.
cosmic microwave background, quadrupole component Quadrupole variation of the temperature pattern of the cosmic microwave background across the sky. It was first measured in 1992 by the Differential Microwave Radiometer (DMR) experiment on board the COBE satellite, launched by NASA in 1989. The cosmic microwave background temperature fluctuations were measured at an angular resolution of 7o at frequencies of 31.5, 53, and 90 GHz. The r.m.s. quadrupole anisotropy amplitude is
defined through Q2rms/To2 = m |a2m|2/4π, with To the cosmic microwave background tem-
perature and a2m the five (l = 2) multipoles of the spherical harmonic expansion of the temperature pattern (see cosmic microwave background temperature anisotropies, Sachs–Wolfe effect). The observed cosmic microwave background quadrupole amplitude is Qrms = 10.7± 3.6±7.1µ K, where the quoted errors reflect the 68% confidence uncertainties from statistical errors and systematic errors associated to the modeling of the galactic contribution, respectively.
More interesting for cosmological purposes is the quadrupole obtained from a power law
fit to the |
entire |
radiation |
power spectrum, |
||||||||
Qrms−P S. |
The data indicates that the spec- |
||||||||||
tral |
index |
of matter density perturbations is |
|||||||||
n |
= |
1.2 |
± 0.3 and the |
quadrupole normaliza- |
|||||||
|
|
|
|
3.8 |
µ K. For n |
|
1, |
||||
tion Q |
rms |
−P S = |
15.3+ |
|
= |
||||||
|
|
|
|
−2.8 |
|
= |
|||||
the best-fit normalization is Qrms−P S|(n=1) |
18 ± 1.6µ K. The difference between the two definitions reflects the statistical uncertainty associated with the large sampling variance of
© 2001 by CRC Press LLC

cosmic microwave background, temperature fluctuations
Qrms since it is obtained from only five independent measurements.
cosmic microwave background, spectral distortions The cosmic microwave background is well characterized by a 2.728 ± 0.002 K black body spectrum over more than three decades in frequency. Spectral distortions could have been produced by energy released by decaying of unstable dark matter particles or other mechanisms. Free-free processes (bremsstrahlung and free-free absorption) become ineffective to thermalize the radiation below redshift zff 105(IB h2)−6/5, where IB is the baryon fraction of the total density in units of the critical density, and h is the dimensionless Hubble constant. Any processes releasing energy later than zff will leave a distinctive imprint on the spectrum of the cosmic microwave background. After zff , Compton scattering between the radiation and electron gas is the only process than can redistribute the photon energy density, but as it conserves photon number, it does not lead to a Planckian spectrum. The lack of any distortion on the cosmic microwave background spectrum sets a very strict upper limit on the fractional energy released in the early universe: ?E/Ecmb < 2 × 10−4 for redshifts between 5 × 106 and recombination. The only distortion detected up to now is a temperature deficit in the direction of clusters of galaxies due to inverse Compton scattering of cosmic microwave background photons by hot electrons in the cluster atmosphere. See Sunyaev–Zeldovich effect.
cosmic microwave background, temperature fluctuations Variation on the cosmic microwave background temperature across the sky. Current observations show that the cosmic microwave background has a dipole anisotropy at the 10−3 level and smaller scale anisotropies at the 10−5 level in agreement with the expectations of the most widely accepted models of structure formation. It is customary to express the cosmic microwave background temperature anisotropies on the sky in a spherical harmonic expansion,
?T |
|
|
(θ, φ) = almYlm(θ, φ) . |
T |
|
|
lm |
The dipole (l = 1) is dominated by the Doppler shift caused by the Earth’s motion relative to the nearly isotropic blackbody field (see cosmic microwave background dipole component). The lower order multipoles (2 ≤ l ≤ 30), corresponding to angular scales larger than the horizon at recombination, are dominated by variations in the gravitational potential across the last scattering surface (see Sachs–Wolfe effect). On smaller angular scales, peculiar motions associated with the oscillation in the baryon-photon plasma dominate the contribution, giving rise to variations in power between l 100 and l 1000 known as Doppler peaks. Together, other physical processes can contribute to increase the intrinsic anisotropies along the photon trajectory such as integrated Sachs–Wolfe, Sunyaev–Zeldovich, Vishniac or Rees–Sciama effects. The pattern of temperature anisotropies and the location and relative amplitude of the different Doppler peaks depend on several cosmological parameters: Hubble constant, baryon fraction, dark matter and cosmological constant contributions to the total energy density, geometry of the universe, spectral index of matter density perturbations at large scales, existence of a background of gravitational waves, etc. In this respect, the cosmic microwave background is an excellent cosmological probe and a useful test of models of galaxy and structure formation. The character of the fluctuations is usually described by the best fitting index n and Qrms−P S, the mean r.m.s. temperature fluctuations expected in the quadrupole component of the anisotropy averaged over all cosmic observers.
A cosmological model does not predict the exact cosmic microwave background temperature that would be observed in our sky, but rather predicts a statistical distribution of anisotropy parameters, such as spherical harmonic amplitudes: Cl =< |alm|2 > where the average is over all cosmic observers. In the context of these models, the true cosmic microwave background temperature observed in our sky is only a single realization from a statistical distribution. If the statistical distribution is Gaussian, and the spectral index of matter density perturbation spectrum is n = 1, as favored by inflation, then Cl = 6C2/l(l+1). The figure displays the mean temperature offset δTl = (l(l + 1)Cl/2π)1/2To of several experiments carried out to measure
© 2001 by CRC Press LLC
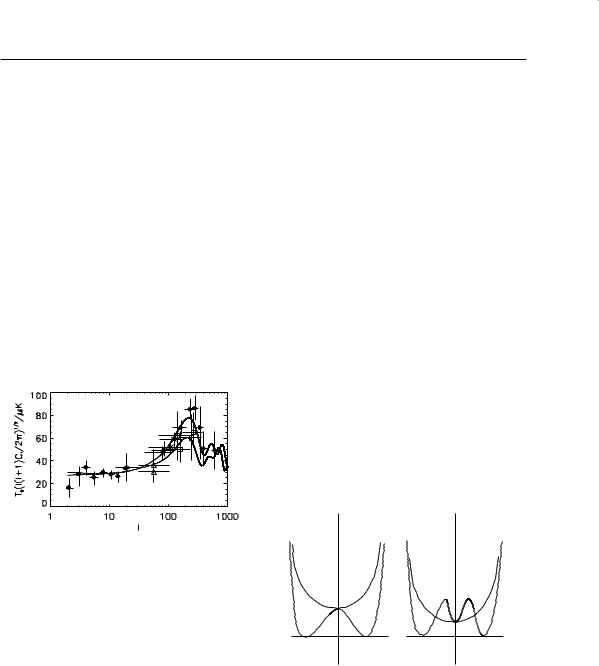
cosmic nucleosynthesis
temperature anisotropies on all angular scales. The data indicate a plateau at l 20, suggesting a spectral index close to n = 1 and a rise from l = 30 to 200, as it would correspond to the first Doppler peak if I = 1. Error bars in the vertical direction give 68% confidence uncertainty. In the horizontal direction indicate the experiments angular sensitivity. We have supersoped the predictions of three flat models: Icdm = 0.95 h = 0.65, Icdm = 0.95, IU = 0.7 and h = 0.7, and Ihdm = 0.95 h = 0.65 to show the agreement between data and theory. In both cases the baryon fraction was IB = 0.05, in units of the critical density. Icdm represents the fraction of cold dark matter, Ihdm of hot dark matter, and IU the contribution of the cosmological constant to the total energy density.
Experimental results as to January 1999. Measurements of anisotropies have been converted into temperature offsets. The predictions of two models are superposed with: cold dark matter (ICDM = 0.95, IB h2 = 0.02, h = 0.65) and cold dark matter with
cosmological constant (to = 14×109 yr, IU = 0.7,
ICDM = 0.25, IB h2 = 0.02).
cosmic nucleosynthesis |
Big Bang nucle- |
osynthesis. |
|
cosmic phase transition |
The idea that phase |
transitions would occur in the early universe, originally borrowed from condensed matter and statistical physics. Examples of such cosmic transitions are the quark to hadron (confinement) phase transition, which quantum chromodynamics (QCD, the theory of strongly interacting particles) predicts at an energy around 1 GeV, and the electroweak phase transition at
about 250 GeV. Within grand unified theories, aiming to describe the physics beyond the standard model, other phase transitions are predicted to occur at energies of order 1015 GeV.
In cosmic phase transitions, the system is the expanding, cooling down universe, and the role of the order parameter is commonly assigned to the vacuum expectation value |φ| of hypothetical scalar Higgs fields (denoted φ in what follows), which characterize the ground state of the theory. In the transition the expected value of the field goes from zero (the high temperature symmetric phase) to a nonvanishing value (in the low temperature broken symmetry phase, which does not display all the symmetries of the Hamiltonian).
The evolution of the order parameter (φ in our case) can be a continuous process (for secondorder transitions). It can also proceed by bubble nucleation or by spinoidal decomposition (firstorder transition), in which case φ changes from zero to its low temperature value abruptly. Typical effective potentials are shown on the figures.
V(φ) |
V(φ) |
T>Tc |
T>Tc |
|
φ |
φ |
T=0 |
T=0 |
a |
b |
Effective potentials used to describe cosmic phase transitions: (a) for a second-order phase transition and (b) for a first-order phase transition. In both cases, the potential has a minimum value for φ = 0 at high temperatures (i.e., T Tc, dotted lines), while its shape is modified at low temperatures (solid lines). First-order transitions are characterized by the fact that there exist two qualitatively distinct types of minima at low temperature, the symmetric phase (φ = 0) being metastable instead of unstable as is the case for a second-order transition.
© 2001 by CRC Press LLC