
Erik_Kandel_-_V_poiskakh_pamyati_angl
.pdf
copulating chains in which each member serves as both male for the animal in front of it and female for the animal behind it in the chain.
As we analyzed and thought about these behaviors, we realized they were all too complex, some involving more than one ganglion of the snail's nervous system. We needed to find a very simple behavior controlled by the cells of one ganglion. We therefore focused on the several behaviors controlled by the abdominal ganglion, the one I had studied in Paris and with which I was most familiar. The abdominal ganglion, which contains only two thousand nerve cells, controls heart rate, respiration, egg laying, inking, release of mucus, and withdrawal of the gill and siphon. In 1968 we settled on the simplest behavior: the gill-withdrawal reflex.
The gill is an external organ that Aplysia uses to breathe. It lies in
13-1 One full step. Aplysia moves by lifting its head and releasing suction to raise the front of its foot, which it then extends for a distance equal to half its body length. The animal brings the front of its foot down, attaches it to a surface, then contracts its anterior end to take up the slack. (Courtesy of Paul Kandel.)
a cavity of the body wall called the mantle cavity and is covered by a sheet of skin called the mantle shelf. The mantle shelf ends in the siphon, a fleshy spout that expels seawater and waste from the mantle cavity (figure 13-3A). Touching the siphon lightly produces a brisk defensive withdrawal of both the siphon and the gill into the mantle cavity (figure 13-3B). The purpose of the withdrawal reflex is clearly to protect the gill, a vital and delicate organ, from possible damage.
Irving and I found that even this very simple reflex can be modified by two forms of learning— habituation and sensitization—and each gave rise to a short-term memory that lasts for a few minutes. An initial light touch to the siphon produces brisk withdrawal of the gill. Repeated light touches produce habituation: the reflex progressively weakens as the animal learns to recognize that the stimulus is trivial. We produced sensitization by applying a strong shock to either the head or the tail. The animal recognized the strong stimu-
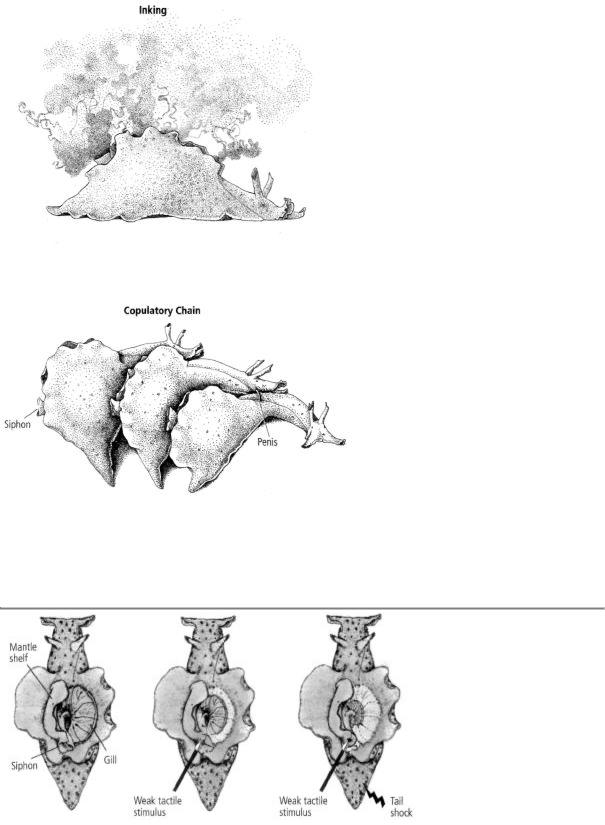
13-2 Simple and complex behaviors in Aplysia. Inking (above) is a relatively simple behavior, controlled by cells in a single ganglion (the abdominal ganglion) of the snail's nervous system. Sexual behavior is far more complex and involves nerve cells in several ganglia. Aplysia are hermaphrodites, able to be both male and female, and often form copulatory chains such as the one shown (below). (Reprinted from Cellular Basis of Behavior, E. R. Kandel, W H. Freeman and Company, 1976.)
A. The gill, through |
B. The gill withdraws into the |
After repetition of a weak |
which Aplysia breathes, |
mantle cavity for protection |
touch on its siphon, the snail |
is normally relaxed. |
when the snail is startled by a becomes habituated to the stim- |

touch on its siphon. Even this |
ulus and its withdrawal reflex |
|
simple response can be modidiminishes. But when the weak fied by habituation, sensitiza- |
touch is paired with a shock to |
|
tion, and classical |
the tail, Aplysia becomes sensi- |
|
conditioning. |
tized and responds with a strong |
|
gill-withdrawal reflex even to the weak touch alone.
13-3 Aplysia's simplest behavior, the gill-withdrawal reflex.
lus as noxious and subsequently produced an exaggerated gill-withdrawal reflex in response to the same light touch to the siphon (figure 13-3C).
In 1971 we were joined by Tom Carew, a gifted, energetic, and gregarious physiological psychologist from the University of California, Riverside, who opened up our study of long-term memory. Carew simply loved being in the neurobiology and behavior group at NYU. He became good friends with Jimmy Schwartz and Alden Spencer as well as with me. Like a dry sponge, Carew soaked up the culture of the group— not only the science, but also the shared interest in art, music, and scientific gossip. As Carew and I would say to each other, "When other people engage in this talk, it's gossip; when we do it, it's intellectual history."
Carew and I found that long-term memory in Aplysia, as in people, requires repeated training interspersed with periods of rest. Practice makes perfect, even in snails. Thus, forty stimuli administered consecutively result in habituation of gill withdrawal that lasts only one day,
but ten stimuli every day for four days produce habituation that lasts for weeks. Spacing the training with periods of rest enhances the ability of an Aplysia to establish long-term memory.
Kupfermann, Carew, and I had demonstrated that a simple reflex was amenable to two nonassociative forms of learning, each with shortand long-term memory. In 1983 we succeeded in reliably producing classical conditioning of the gill-withdrawal reflex. This was an important advance since it demonstrated that the reflex can also be modified by associative learning.
By 1985, after more than fifteen years of hard work, we had shown that a simple behavior in Aplysia could be modified by various forms of learning. This strengthened my hope that some forms of learning had been conserved throughout evolution and would be found even in a simple neural circuit of a very simple behavior. Moreover, I could now foresee the possibility of going beyond the questions of how learning occurs and how memory is stored in the central nervous system to the question of how different forms of learning and memory relate to each other at the cellular level. Specifically, how is short-term memory converted to long-term memory in the brain?
BEHAVIORAL STUDIES OF THE GILL-WITHDRAWAL REFLEX WERE
not the sole focus of our work during this time. Indeed, they formed the groundwork for our second and principal interest—devising experiments to explore what happens in the brain of an animal as it learns. Thus, once we had decided to focus our study of learning on the gill-withdrawal reflex in Aplysia, we needed to map the neural circuitry of the reflex to learn how the abdominal ganglion brings it about.
Working out the neural circuitry posed its own conceptual challenge. How precise and specialized are the connections among the cells of a neural circuit? In the early 1960s some followers of Karl Lashley argued that the properties of the different neurons in the cerebral cortex are so similar that they are to all intents and purposes identical, and their interconnections random and of roughly equal value.
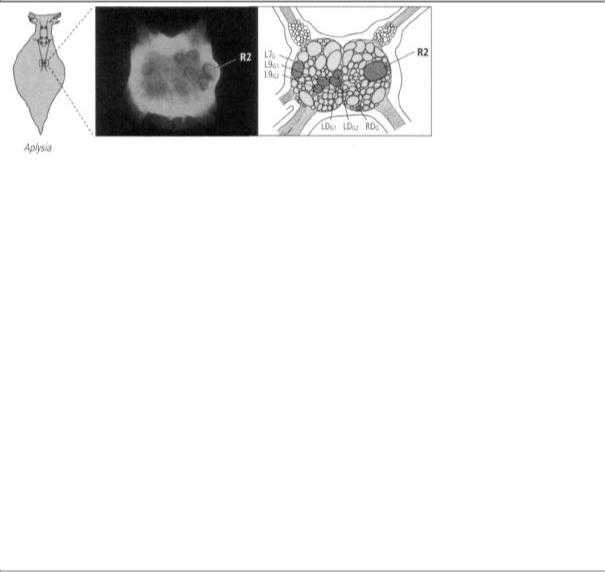
Other scientists, particularly students of the invertebrate nervous system, championed the idea that many, perhaps all, neurons are unique. This idea was first proposed in 1908 by the German biologist
13-4 Identifying specific neurons in the abdominal ganglion of Aplysia. Cell R2 can be clearly seen in a photomicrogaph (left) of Aplysia's abdominal ganglion. It measures 1 millimeter in diameter. A drawing (right) shows the position of cell R2 and the six motor neutrons controlling movement of the gill. Once individual neurons had been identified, it was possible to map their connections.
Richard Goldschmidt. Goldschmidt studied a ganglion of the nematode worm Ascaris, a primitive intestinal parasite. He found that every animal of the species has the same number of cells in exactly the same position in that ganglion. In a now famous lecture to the German Zoological Association that year, he noted, "the almost startling constancy of the elements of the nervous system: There are 162 ganglion cells in the center, never one more nor less."
Angélique Arvanitaki-Chalazonitis was aware of Goldschmidt's analysis of the worm, and in the 1950s she explored the abdominal ganglion of Aplysia in search of identifiable cells. She found that several cells are unique and identifiable in every individual animal on the basis of their location, pigmentation, and size. One such cell was R2, the cell I had focused on in my studies of learning with Ladislav Tauc. First at Harvard and later at NYU, I followed up on this lead, and by 1967 I had found, as had Goldschmidt and Arvanitaki-Chalazonitis earlier, that I could easily identify most of the prominent cells in the ganglion (figure 13-4).
The finding that neurons are unique and that the same cell appears in the same location in every member of the species led to new questions: Are the synaptic connections between these unique neurons also invariant? Does a given cell always signal exactly the same target cell and not others?
To my surprise, I found that I could readily map the synaptic connections between cells. By inserting a microelectrode into a target cell and stimulating action potentials in other cells of the ganglion, one cell at a time, I could identify many of the presynaptic cells that communicate with the target cell. Thus it proved possible for the first time in any animal to map the working synaptic connections between individual cells, which I could use as a method for working out the neural circuit controlling a behavior.
I found the same specificity of connections between individual neurons that Santiago Ramón y Cajal had found between populations of neurons. What's more, just as neurons and their synaptic connections are exact and invariant, so, too, the function of those connections is invariant. This extraordinary invariance would make it easier for me to realize my long-term goal of "trapping" learning in a simple set of neural connections in order to look at how learning gives rise to memory at the cellular level.
By 1969 Kupfermann and I had succeeded in identifying most of the nerve cells that make up the gill-withdrawal reflex. To do this we briefly anesthetized the animal so that we could make a small incision in its neck, and then gently lifted the abdominal ganglion and its attached nerves out through the opening and put them on an illuminated stage. We inserted into various neurons the double-barreled microelectrodes we used for recording and stimulating a cell. Opening up the living animal in this way allowed us to keep its nervous system and all its
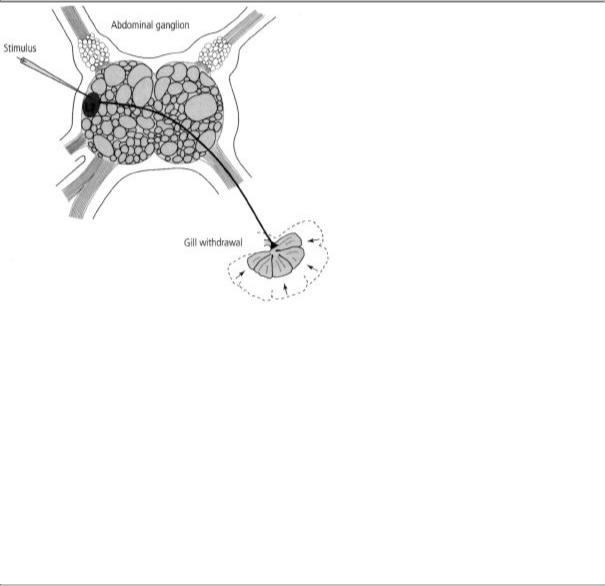
normal connections intact and thus to observe all of the organs controlled by the abdominal ganglion at the same time. We first set about searching for the motor neurons that control the gill-withdrawal reflex—that is, the motor cells whose axons lead outward from the central nervous system to the gill. We did this by stimulating one cell at a time with the microelectrode and watching to see if that stimulus produced a movement of the gill.
One afternoon in the fall of 1968, working alone, I stimulated a cell and was astonished to see that it produced a powerful contraction of the gill (figure 13-5). For the first time I had identified a motor neuron in Aplysia that controlled a specific behavior! I could hardly wait to show
13-5 Discovering a motor neuron that produces a specific behavior in Aplysia. Once the individual nerve cells in Aplysia's abdominal ganglion had been identified, it became possible to map their connections. For example, stimulating cell L7 (a motor neuron) produces a sudden contraction of the animal's gill.
Irving. We both were amazed to see the powerful behavioral consequences of stimulating a single cell and knew it boded well for identifying other motor cells. Indeed, within a few months Irving had found five other motor cells. We suspected that those six cells accounted for the motor component of the gill-withdrawal reflex because if we prevented the cells from firing, no reflex response occurred.
In 1969 I was joined by Vincent Castellucci, a delightful and highly cultivated Canadian scientist with an extensive background in biology who regularly trounced me in tennis, and by Jack Byrne, a technically gifted graduate student with training in electrical engineering who brought the rigor of that discipline to bear on our joint work. Together, the three of us identified the sensory neurons of the gillwithdrawal reflex. We then discovered that in addition to their direct connections,
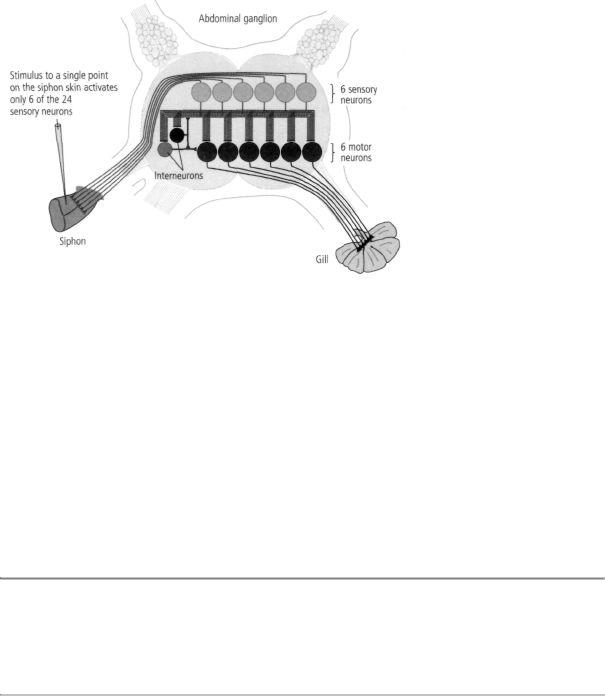
13-6 The neural architecture of Aplysia's gill-withdrawal reflex. The siphon system has 24 sensory neurons, but a stimulus applied to any one point on the skin activates only 6 of them. The same 6 sensory neurons relay the sensation of touch to the same 6 motor neurons in every snail, producing the gill-withdrawal reflex.
the sensory neurons formed indirect synaptic connections with motor neurons through interneurons, a type of intermediary neuron. Those two sets of connections—the direct and indirect—relay information about touch to the motor neurons, which actually produce the withdrawal reflex by means of their connections with gill tissue. Moreover, the same neurons were involved in the gill-withdrawal reflex in every snail we studied, and the same cells always formed the same connections with one another. Thus, the neural architecture of at least one behavior of Aplysia was amazingly precise (Figure 13-6). In time, we found the same specificity and invariance in the neural circuitry of other behaviors.
Kupfermann and I ended our 1969 paper in Science, "Neuronal Controls of a Behavioral Response Mediated by the Abdominal Ganglion of Aplysia," on an upbeat note:
In view of its advantages for cellular neurophysiological studies, this preparation may prove useful for analyzing the neuronal mechanisms of learning. Initial experiments indicate that the behavioral reflex response can be modified to show simple learning such as sensitization, habituation.... It may also prove possible to study more complex behavioral modifications using either classical or operant conditioning paradigms.
14
SYNAPSES CHANGE WITH EXPERIENCE
Once we had determined that the neural architecture of a behavior is invariant, we were faced with a critical question: How can a behavior that is controlled by a precisely wired neural circuit be changed through experience? One solution had been proposed by Cajal, who suggested that learning could change the strength of the synapses between neurons, thereby strengthening communication between them. Interestingly, Freud's "Project for a Scientific Psychology" outlines a neural model of mind that includes a similar mechanism of learning. Freud postulated that there are separate sets of neurons for perception and memory. The neural circuits concerned with perception form synaptic connections that are fixed, thus assuring the accuracy of our perceptual
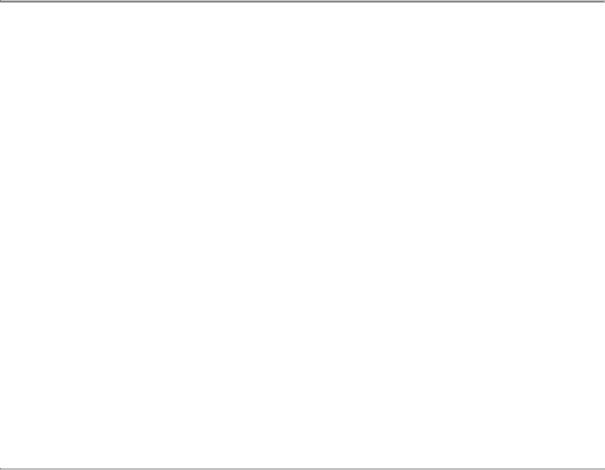
world. The neural circuits concerned with memory have synaptic connections that change in strength with learning. This mechanism forms the basis of memory and higher cognitive functioning.
The work of Pavlov and the behaviorists and that of Brenda Milner and the cognitive psychologists had led me to the realization that different forms of learning give rise to different forms of memory. I had therefore reformulated Cajal's idea and used that new insight as a basis for developing analogs of learning in Aplysiü. The results of that work
had shown that different patterns of stimulation alter the strength of synaptic connections in different ways. But Tauc and I had not examined how an actual behavior is changed and therefore had no evidence that learning really relies on changes in synaptic strength.
Indeed, the very idea that synapses could be strengthened by learning and thus contribute to memory storage was by no means generally accepted. Two decades after Cajal's proposal, the distinguished Harvard physiologist Alexander Forbes suggested that memory is maintained by dynamic, ongoing changes within a closed loop of self-exciting neurons. To support this idea, Forbes cited a drawing by Rafael Lorente de Nó, a student of Cajal, which showed that neurons connect to each other in closed pathways. The idea was further elaborated by the psychologist D. O. Hebb in his influential 1949 book, The Organization of Behavior: A Neuropsychological Theory. Hebb argued that reverberatory circuits are responsible for short-term memory.
Similarly, B. Delisle Burns, a leading student of the biology of the cerebral cortex, challenged the idea that physical changes in synapses can serve as a means of memory storage:
The mechanisms of synaptic facilitation which have been offered as candidates for an explanation of memory . . .
have proven disappointing. Before any of them can be accepted as the cellular changes accompanying conditioned reflex formation, one would have to extend considerably the scale of time on which they have been observed to operate. The persistent failure of synaptic facilitation to explain memory makes one wonder whether neurophysiol-ogists have not been looking for the wrong kind of mechanisms.
Some scholars questioned whether learning could take place at all in fixed neural circuits. For them, learning had to be partially or even totally independent of preestablished neuronal pathways. This view was held by Lashley and by some members of an influential group of early cognitive psychologists, the Gestalt psychologists. A variant of this idea was put forward in 1965 by the neurophysiologist Ross Adey. He began his argument by saying that "no neuron in natural or artificial isolation from other neurons has been shown capable of storing
information in the usual notion of memory." He then went on to argue that the flow of current through the space between neurons may carry information that ranks "at least equivalently with neuronal firing in the transaction of information and even more importantly in its deposition and recall." For Adey as for Lashley learning was completely mysterious.
Having worked out the neural circuitry of the gill-withdrawal reflex and determined that it could be modified by learning, my colleagues and I were in a position to ask which, if any, of these ideas had merit. In the first of three consecutive papers we published in the journal Science in 1970, we outlined the research strategy that we had used and that was to guide our thinking for the next three decades:
The analysis of the neural mechanisms of learning and similar behavioral modifications requires an animal whose behavior is modifiable and whose nervous system is accessible for cellular analysis. In this and the subsequent two papers, we have applied a combined behavioral and cellular neurophysiological approach to the marine mollusk Aplysia in order to study a behavioral reflex that undergoes habituation and dishabituation (sensitization). We have progressively simplified the neural circuit of this behavior so that the action of individual neurons could be related to the total reflex. As a result, it is now possible to analyze the locus and the mechanisms of these behavioral modifications.
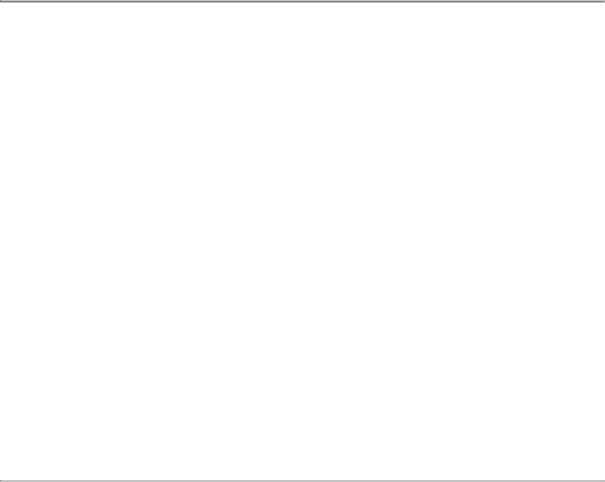
In the ensuing papers we established that memory does not depend on self-exciting loops of neurons. For the three simple forms of learning we studied in Aplysia, we found that learning leads to a change in the strength of synaptic connections—and therefore in the effectiveness of communication—between specific cells in the neural circuit that mediates the behavior.
Our data spoke clearly and dramatically. We had delineated the anatomical and functional workings of the gillwithdrawal reflex by recording from individual sensory and motor neurons. We had found that touching the skin activates several sensory neurons that together produce a large signal—a large synaptic potential—in each of the
motor neurons, causing them to fire several action potentials. These action potentials in the motor neurons produce a behavior—the withdrawal of the gill. We could see that under normal circumstances, the sensory neurons communicate effectively with the motor neurons, sending them an adequate signal to produce the gillwithdrawal reflex.
We now turned our attention to the synapses between the sensory and motor neurons. We observed that when we produced habituation by touching the skin repeatedly, the amplitude of the gill-withdrawal reflex decreased progressively. This learned change in behavior was paralleled by a progressive weakening of the synaptic connections. Conversely, when we produced sensitization by applying a shock to the animal's tail or head, the enhanced gill-withdrawal reflex was accompanied by a strengthening of the synaptic connection. We concluded that during habituation an action potential in the sensory neuron gives rise to a weaker synaptic potential in the motor neuron, leading to less effective communication, while during sensitization it gives rise to a stronger synaptic potential in the motor neuron, leading to more effective communication.
In 1980 we carried our reductionist approach one step further and explored what happens at the synapses during classical conditioning. Carew and I were joined in this endeavor by Robert Hawkins, an insightful young psychologist from Stanford University. The son of an academic family, he did not need New York to broaden his horizons: he was already a devotee of classical music and opera. A fine athlete, Hawkins had played on the varsity soccer team at Stanford, and he proceeded to focus his athletic passion on sailing.
We found that in classical conditioning, the neural signals from the innocuous (conditioned) and noxious (unconditioned) stimuli must occur in a precise sequence. That is, when the siphon is touched just before the tail is—thus predicting the shock to the tail—the sensory neurons will fire action potentials just before they receive signals from the tail. The precisely timed firing of action potentials in the sensory neurons, followed by the precisely timed arrival of the signals from the tail shock, leads to much greater strengthening of the synapse between the sensory and motor neurons than when signals from the siphon or the tail occur separately, as they do in sensitization.
These several results on habituation, sensitization, and classical conditioning led us irresistibly to think about how genetic and developmental processes interact with experience to determine the structure of mental activity. Genetic and developmental processes specify the connections among neurons—that is, which neurons form synaptic connections with which other neurons and when. But they do not specify the strength of those connections. Strength—the long-term effectiveness of synaptic connections—is regulated by experience. This view implies that the potential for many of an organism's behaviors is built into the brain and is to that extent under genetic and developmental control; however, a creature's environment and learning alter the effectiveness of the preexisting pathways, thereby leading to the expression of new patterns of behavior. Our findings in Aplysia supported this view: in its simplest forms, learning selects among a large repertoire of preexisting connections and alters the strength of a subset of those connections.
In reviewing our results, I could not help being reminded of the two opposing philosophical views of mind that had dominated Western thought from the seventeenth century onward—empiricism and rationalism. The British empiricist John Locke argued that the mind does not possess innate knowledge but is instead a blank slate that is eventually filled by experience. Everything we know
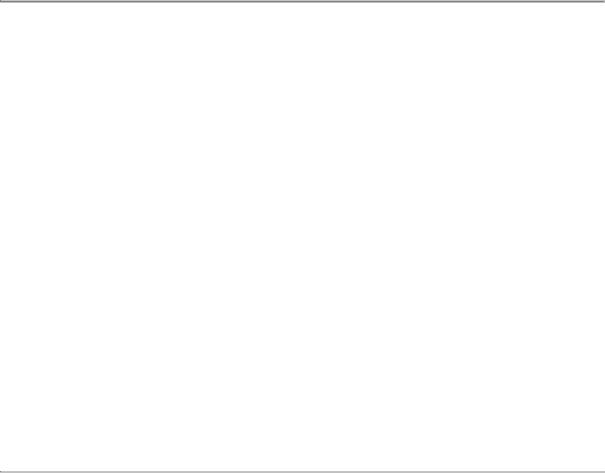
about the world is learned, so the more often we encounter an idea, and the more effectively we associate it with other ideas, the more enduring its impact on our minds. Immanuel Kant, the German rationalist philosopher, argued to the contrary, that we are born with certain built-in templates of knowledge. Those templates, which Kant called a priori knowledge, determine how sensory experience is received and interpreted.
In choosing between psychoanalysis and biology as a career, I had decided on biology because psychoanalysis, and its predecessor discipline, philosophy, treated the brain as a black box, an unknown. Neither field could resolve the conflict between the empiricist and rationalist views of mind as long as the resolution required a direct examination of the brain. But examining the brain was just what we had begun to do. In the gill-withdrawal reflex of this simplest of
organisms, we saw that both views had merit—in fact, they complemented each other. The anatomy of the neural circuit is a simple example of Kantian a priori knowledge, while changes in the strength of particular connections in the neural circuit reflect the influence of experience. Moreover, consistent with Locke's notion that practice makes perfect, the persistence of such changes underlies memory.
Whereas the study of complex learning had seemed intractable to Lashley and others, the elegant simplicity of the gill-withdrawal reflex in a snail enabled my colleagues and me to address experimentally a number of the philosophical and psychoanalytical questions that had led me to biology in the first place. This I found to be both amazing and humorous.
In the third of our reports in Science in 1970, we concluded with these comments:
[T]he data indicate that habituation and dishabituation (sensitization) both involve a change in the functional effectiveness of previously existing excitatory connections. Thus, at least in the simple cases, . . . [t]he capability for behavioral modification seems to be built directly into the neural architecture of the behavioral reflex.
Finally, these studies strengthen the assumption . . . that a prerequisite for studying behavioral modification is the analysis of the wiring diagram underlying the behavior. We have, indeed, found that once the wiring diagram of the behavior is known, the analysis of its modification becomes greatly simplified. Thus, although this analysis pertains to only relatively simple and short-term behavioral modifications, a similar approach may perhaps also be applied to more complex as well as longer lasting learning processes.
By sticking with a radically reductionist approach—examining a very simple behavioral reflex and simple forms of learning, delineating cell by cell the neural circuit of the reflex, and then focusing on where change occurs within that circuit—I had reached the long-term goal outlined in my grant application to NIH in 1961. I had "trapped a
conditioned response in the smallest possible neural population, the connections made between two cells."
THUS THE REDUCTIONIST APPROACH LED US TO DISCOVER
several principles of the cell biology of learning and memory. First, we found that the changes in synaptic strength that underlie the learning of a behavior may be great enough to reconfigure a neural network and its information-processing ability. For example, one particular sensory cell in Aplysia communicates with eight different motor cells—five that produce movement of the gill and three that cause contraction of the ink gland and thus inking. Before training, activation of this sensory cell excited the five gill-innervating motor cells moderately, causing them to fire action potentials and thereby causing the gill to contract. Activation of this same sensory cell also excited the three ink
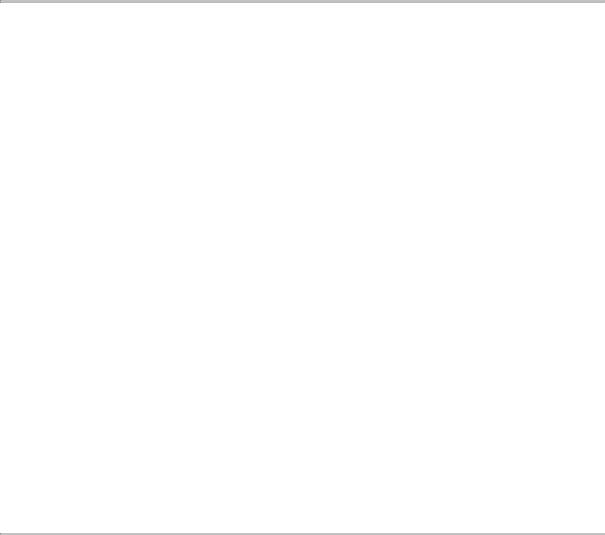
gland-innervating motor neurons but only very weakly, not enough to produce action potentials or to elicit inking. Thus, before learning, gill withdrawal would take place in response to stimulation of the siphon but inking would not. After sensitization, however, synaptic communication between the sensory cell and all eight motor cells is enhanced, causing the three ink gland-innervating motor neurons to fire action potentials as well. Thus, as a result of learning, when the siphon is stimulated, inking will occur along with more powerful gill withdrawal.
Second, consistent with my reformulation of Cajal's theory and my earlier work with analogs, we found that a given set of synaptic connections between two neurons can be modified in opposite ways— strengthened or weakened—by different forms of learning. Thus, habituation weakens the synapse, whereas sensitization or classical conditioning strengthens it. These enduring changes in the strength of synaptic connections are the cellular mechanisms underlying learning and short-term memory. Moreover, because the changes occur at several sites in the neural circuitry of the gillwithdrawal reflex, memory is distributed and stored throughout the circuit, not at a single specialized site.
Third, we found that in all three forms of learning, the duration of
short-term memory storage depends on the length of time a synapse is weakened or strengthened.
Fourth, we were beginning to understand that the strength of a given chemical synapse can be modified in two ways, depending on which of two neural circuits is activated by learning—a mediating circuit or a modulatory circuit. In Aplysia, the mediating circuit is made up of the sensory neurons that innervate the siphon, the interneurons, and the motor neurons that control the gillwithdrawal reflex. The modulatory circuit is made up of sensory neurons that innervate the tail in a completely different part of the body. When the neurons in a mediating circuit are activated, homosynaptic changes in strength occur. This is the case in habituation: the sensory and motor neurons that control the gill-withdrawal reflex fire repeatedly and in a certain pattern in direct response to the repeated sensory stimulus. Heterosynaptic changes in strength occur when the neurons in a modulatory, rather than the mediating, neural circuit are activated. This is the case with sensitization: the strong stimulus to the tail activates a modulatory circuit that controls the strength of synaptic transmission in the mediating neurons.
We later found that classical conditioning recruits both homosynaptic and heterosynaptic changes. Indeed, our studies of the relationship of sensitization to classical conditioning indicate that learning may be a matter of combining various elementary forms of synaptic plasticity into new and more complex forms, much as we use an alphabet to form words.
I now began to realize that the abundance of chemical over electrical synapses in the brains of animals may reflect a fundamental advantage of chemical over electrical transmission: the ability to mediate a variety of forms of learning and of memory storage. Viewed from this perspective, it became clear that the synapses between sensory neurons and motor neurons in the gill-withdrawal circuit—neurons that have evolved to participate in various types of learning—are much more easily changed than synapses that play no role in learning. Our studies showed dramatically that in circuits modified by learning, synapses can undergo large and enduring changes in strength after only a relatively small amount of training.