
Tools and Applications of Biochemical Engineering Science
.pdf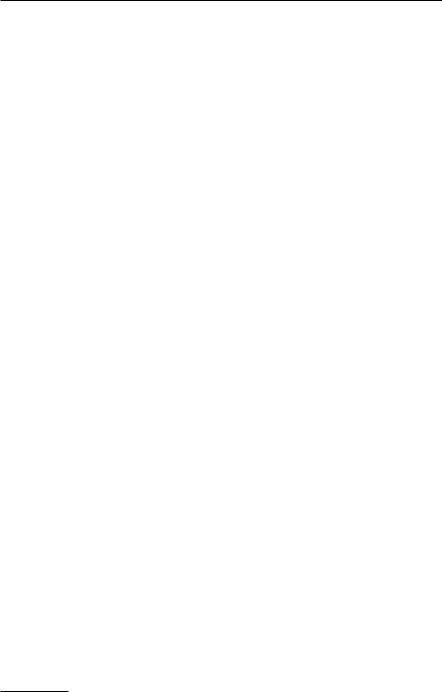
Cell Retention Devices for Suspended-Cell Perfusion Cultures
Leda R. Castilho1,*, Ricardo A. Medronho2
1GBF-German National Research Center for Biotechnology, Biochemical Engineering Division, Mascheroder Weg 1, 38124 Braunschweig, Germany
E-mail: ledacastilho@ieg.com.br
2Federal University of Rio de Janeiro, Department of Chemical Engineering, School of Chemistry, Centro de Tecnologia, Bloco E, Ilha do Fundão, 21949-900 Rio de Janeiro – RJ, Brazil
E-mail: medronho@ufrj.br
Dedicated to Prof. Dr. Wolf-Dieter Deckwer on the occasion of his 60th birthday
Perfusion cultures of animal cells have several advantages over batch or fed-batch cultures. They give, for instance, higher productivities and a consistent product quality, and allow steady state operation and better cell physiology control. However, one of the main aspects limiting performance and scale-up of perfusion processes is the need for an adequate cell retention device. The devices currently in use for stirred perfusion bioreactors are continuous centrifuges, tangential flow membrane filters, dynamic filters, spin-filters, ultrasonic and dielectrophoretic separators, gravity settlers and, more recently, hydrocyclones. The advantages and disadvantages of each of these methods will be discussed.
Keywords. Perfusion cultures, mammalian cell retention devices, filtration, centrifugation, sedimentation
1 |
Introduction . . . . . . . . . . . . . . . . . . . . . . . . . . . . . . . . |
131 |
2 |
Centrifugation . . . . . . . . . . . . . . . . . . . . . . . . . . . . . . . |
134 |
3 |
Hydrocyclones . . . . . . . . . . . . . . . . . . . . . . . . . . . . . . . |
140 |
4 |
Gravitational Settling . . . . . . . . . . . . . . . . . . . . . . . . . . . |
143 |
5 |
Spin-Filters . . . . . . . . . . . . . . . . . . . . . . . . . . . . . . . . . |
147 |
6 |
Tangential Flow Filtration . . . . . . . . . . . . . . . . . . . . . . . . . |
153 |
7 |
Dynamic Filtration . . . . . . . . . . . . . . . . . . . . . . . . . . . . . |
156 |
8 |
Ultrasonic Separation . . . . . . . . . . . . . . . . . . . . . . . . . . . |
161 |
9 |
Dielectrophoretic Separation . . . . . . . . . . . . . . . . . . . . . . . |
164 |
10 |
Conclusions . . . . . . . . . . . . . . . . . . . . . . . . . . . . . . . . . |
165 |
References . . . . . . . . . . . . . . . . . . . . . . . . . . . . . . . . . . . . |
165 |
*Current address:
Federal University of Rio de Janeiro, COPPE-Chemical Engineering Program, Centro de Tecnologia, sala G-116, 21945-970 Rio de Janeiro/RJ, Brazil
Advances in Biochemical Engineering/
Biotechnology, Vol. 74
Managing Editor: Th. Scheper
© Springer-Verlag Berlin Heidelberg 2002
130 |
L.R. Castilho · R.A. Medronho |
Abbreviations
Aarea (m2)
bfield force intensity (m s–2)
dcell diameter (m)
Dperfusion rate (s–1)
dbioreactor |
bioreactor diameter (m) |
dc |
cut size (m) |
dhf |
hollow fiber internal diameter (m) |
dsf |
spin-filter diameter (m) |
Ecell separation efficiency (–)
E¢ |
reduced total efficiency (–) |
ggravity acceleration (m s–2)
hbioreactor |
bioreactor height (m) |
hsf |
spin-filter height (m) |
Jp |
perfusion flux (m s–1) |
kratio of particle radius to channel width (–)
Qfeed volumetric flow rate (m3 s–1)
Qo |
overflow volumetric flow rate (m3 s–1) |
Qu |
underflow volumetric flow rate (m3 s–1) |
rcell radius (m)
Rradial position of the particle (m)
rcf |
ratio of permeation drag to lift drag in cross-flow filtration (–) |
Re |
Reynolds number (–) |
Rf |
flow ratio (–) |
rsf |
ratio of permeation drag to lift drag in spin-filters (–) |
ttime (s)
Vbioreactor volume (m3)
vp |
velocity due to perfusion flux (m s–1) |
vpermeation |
permeation velocity (m s–1) |
vt |
terminal settling velocity (m s–1) |
vtang |
tangential velocity (m s–1) |
Xcell concentration in the feed suspension (m–3)
Xo |
cell concentration in the overflow (m–3) |
Xu |
cell concentration in the underflow (m–3) |
bcell passage factor (–)
eporosity (–)
hliquid viscosity (Pa s)
mspecific growth rate (s–1)
mapp |
apparent specific growth rate (s–1) |
Çdensity of the liquid (kg m–3)
Çs |
density of the cells (kg m–3) |
wangular velocity (s–1)
Cell Retention Devices for Suspended-Cell Perfusion Cultures |
131 |
1 Introduction
Mammalian cells are commonly employed for the production of therapeutic and diagnostic proteins, since they are able to correctly synthetize the large and complex structures that the human body requires as medicine [1]. Nowadays, they are employed for the large-scale production of recombinant therapeutic proteins, monoclonal antibodies (MAbs) and viruses used in the preparation of vaccines (e.g. against rabies, hepathytis B, polio, etc) [2]. An overview of some licensed/approved products derived from mammalian cell culture is given in Table 1.
Although the initial focus was on diagnostic products, the emphasis in animal cell biotechnology has been increasingly shifting to therapeutic proteins [3]. Furthermore, among the newer products, especially MAbs, a visible trend is that doses are becoming larger. While doses of the earlier rDNA products were in the sub-milligram range, nowadays various new products are administered in tens or hundreds of milligrams [4]. These facts not only enhance the importance of process quality and higher purity levels, but also imply a continuing search for even more efficient ways to grow cells, express and purify proteins. Besides physiological studies and medium optimization, further development of engineering aspects of production systems is a fundamental key for manufacturers to improve productivity and thus cope with the kilogram quantities of product needed nowadays.
Commercial scale cultivation of mammalian cells is accomplished using different technologies: roller bottles, microcarriers, suspension (batch, fed-batch or perfusion mode) and hollow fiber bioreactors (Table 2). However, especially for products needed in large amounts, suspension cultivation seems to be the most effective system [4, 5]. Suspension-based systems are characterized by a homogeneous concentration of cells, nutrients, metabolites and product, thereby facilitating scale-up [6] and enabling an accurate monitoring and control of the culture [7].
Suspension systems can be operated in different modes: batch, fed-batch, chemostat, and perfusion (Fig. 1). These operation modes differ basically in the way nutrient supply and metabolite removal are accomplished, which in turn determines cell concentration, product titer and volumetric productivity that can be achieved [8]. The intrinsic limitation of batch processes, where cells are exposed to a constantly changing environment, limits full expression of growth and metabolic potentials. This aspect is partially overcome in fed-batch cultures, where a special feeding strategy prolonges the culture and allows an increase in cell concentration to be achieved. In perfusion and chemostat processes nutrients are continuously fed to the bioreactor, while the same amount of spent medium is withdrawn. However, in perfusion cultures the cells are retained within the bioreactor, as opposed to continuous-flow culture (chemostat), which washes cells out with the withdrawn medium [9].
Batch, fed-batch and perfusion processes are commonly used in large-scale cultivation of mammalian cells. Chemostat cultures are a valuable tool for cell physiology studies but not a serious contender as a production process [3].
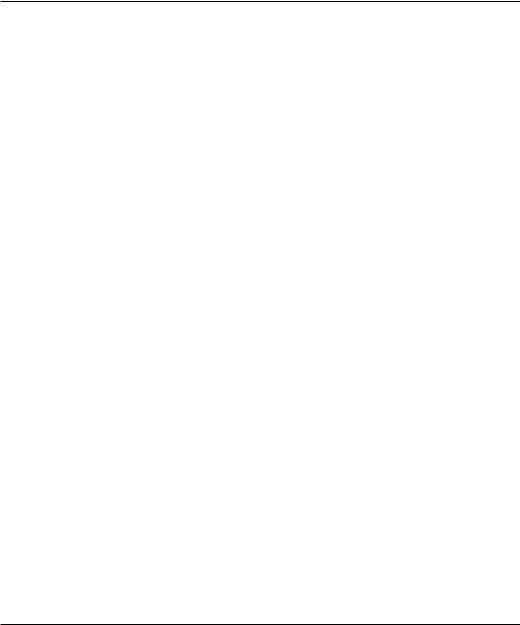
132 |
L.R. Castilho · R.A. Medronho |
Table 1. Some licensed/approved biologicals produced by mammalian cells [2, 4, www.fda.gov/cber/cberac.htm]
Product |
Protein or |
Indication |
Cells |
First |
Countries |
|
MAb/immunogen |
|
|
approval |
|
|
|
|
|
|
|
Activase |
t-PA |
acute ischemic stroke |
CHO |
1987 |
various |
Avonex |
b-interferon |
multiple sclerosis |
CHO |
1996 |
USA |
Bene Fix |
factor IX |
hemophilia B |
CHO |
1997 |
USA |
Cerezyme |
glucocere- |
Gaucher disease |
CHO |
1994 |
USA, Austria, |
|
broxidase |
|
|
|
New Zealand |
Enbrel/Etanercept |
fusion protein |
rheumatoid arthritis |
CHO |
1998 |
USA |
|
(TNFR/hIgG1) |
|
|
|
|
Epogen/Procrit |
EPO |
anemia |
CHO |
1989 |
various |
Epogin/Recormon |
EPO |
anemia |
CHO |
1990 |
Japan, Europe |
GenHevac B Pasteur |
HBsAg |
hepatitis |
CHO |
1989 |
France |
Gonal-F |
FSH |
female infertility |
CHO |
1995 |
Sweden, |
|
|
|
|
|
Finland, USA |
Granocyte |
g-CSF |
anemia, neutropenia |
CHO |
1991 |
Japan, Europe |
HB Gamma |
HBsAg |
hepatitis |
CHO |
1990 |
Japan |
Herceptin/ |
MAb/HER2 |
metastatic breast cancer |
CHO |
1998 |
USA |
Trastuzumaba |
MAb/TFNa |
|
|
|
|
Infliximab/ |
Crohn’s disease |
n.a. |
1998 |
USA |
|
Remicadea,b |
|
|
|
|
|
Kogenateb |
factor VIII |
hemophilia A |
BHK |
1993 |
various |
MAbs for in vitro |
about 100 |
various |
various |
from 1980 |
various |
diagnostic |
different |
|
|
|
|
Myoscint c |
MAb/myosin |
cardiac imaging agent |
n.a. |
1989 |
Europe, USA |
Novo Seven |
factor VIIa |
hemophilia A and B |
BHK |
1996 |
Switzerland, |
Panorexa |
|
|
|
|
Europe, USA |
MAb/n.a. |
colorectal cancer |
n.a. |
1995 |
Germany |
|
Prosta Scintc |
MAb/PSMA |
prostate cancer |
n.a. |
1996 |
USA |
Pulmozyme |
DNAse I |
cystic fibrosis |
CHO |
1993 |
Sweden, USA, |
|
|
|
|
|
Switzerland |
Puregon |
FSH |
female infertility |
n.a. |
1996 |
Denmark |
Recombinate |
factor VIII |
hemophilia A |
CHO |
1992 |
various |
Refacto |
antihemophilic |
hemophilia A |
CHO |
2000 |
USA |
ReoProa |
factor |
|
|
|
|
MAb/Platelet |
cardiac schemic |
n.a. |
1994 |
USA |
|
Rituxana |
IIb/IIIa |
complications |
|
|
|
MAb/CD20 |
B cell non-Hodgkin’s |
CHO |
1997 |
USA |
|
|
MAb/IL2Ra |
lymphoma |
|
|
|
Simulect/ |
acute kidney transplant |
mouse |
1998 |
USA |
|
Basiliximaba |
|
rejection |
myeloma |
|
|
TNKase/ |
t-PA |
acute myocardial |
CHO |
2000 |
USA |
Tenecteplase |
|
infarction |
|
|
|
Verlumac |
MAb/n.a. |
small cell lung cancer |
n.a. |
1996 |
USA |
Wellferon |
interferon a-N1 |
hepatitis C |
human |
1999 |
USA |
|
|
|
lympho- |
|
|
|
|
|
blastoid |
|
|
n.a.: Information not available.
a Therapeutic monoclonal antibody.
b Produced by continuous perfusion process. c Monoclonal antibody for in vivo diagnostic.
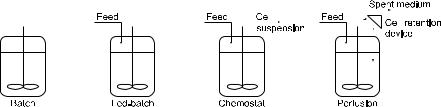
Cell Retention Devices for Suspended-Cell Perfusion Cultures |
133 |
|||||||||
Table 2. Technologies used for commercial-scale cultivation of mammalian cells [4] |
|
|||||||||
|
|
|
|
|
|
|
|
|
|
|
Technology |
Product(s) |
|
||||||||
|
|
|
|
|
|
|
|
|
|
|
Roller bottles |
EPO, h-GH, HbsAg |
|
||||||||
Microcarriers |
glucocerebrosidase |
|
||||||||
Suspension |
t-PA, factor VIII, factor IX, a interferon, several MAbs |
|
||||||||
Perfused suspension |
factor VIII, several MAbs |
|
||||||||
Hollow fiber |
in vivo diagnostic MAb |
|
||||||||
|
|
|
|
|
|
|
|
|
|
|
|
|
|
|
|
|
|
|
|
|
|
|
|
|
|
|
|
|
|
|
|
|
|
|
|
|
|
|
|
|
|
|
|
|
|
|
|
|
|
|
|
|
|
|
|
|
|
|
|
|
|
|
|
|
|
Fig. 1. Schematic view of the different operation modes of suspended-cell processes: batch, fed-batch, chemostat and perfusion
Kadouri and Spier [10] presented the various factors that should be considered before choosing a particular operation mode and proposed a decision tree to facilitate the choice between batch and perfusion processes.
Perfusion is not a modern technique, since it was already employed in 1912 [11] to keep small pieces of tissue viable for extended microscopic observations. It was a result of the observation that in vivo cells are continuously supplied with blood, lymph or another body fluid to keep them in a constant physiological environment, supporting cell concentrations as high as 109 cells/mL [9].
By using a perfusion system, in situ medium exchange is accomplished, and a better control of the culture environment (dissolved oxygen tension, pH, substrate concentration) is possible. Additionally, toxic by-products are removed and secreted product is continuously recovered, avoiding the risk of product degradation [12]. The fresh nutrient supply and metabolites removal, combined with the retention of cells in the bioreactor, allows a high cell concentration to be achieved. This usually results in high product titers even when working at relatively high medium throughput rates, leading to productivities which have been reported to be 10-fold greater than in batch and fed-batch processes [13, 14]. Moreover, perfusion-based processes can produce large volumes of product from a size-limited bioreactor on a continuous basis for extended periods of time [5], reducing capital costs. Additionally, the self-conditioning ability of cells and the less reactor turnover observed in perfusion cultures contribute to reduced operating costs. The self-conditioning ability of cells results from the secretion of growth factors and other proteins by the cells and seems to increase with cell concentration [15], allowing the reduction or complete removal of expensive medium components (e.g. serum) throughout a perfusion cultivation. Less reactor turnover leads to a reduction in labor and energy costs [16].
134 |
L.R. Castilho · R.A. Medronho |
Other advantages related to perfusion operation are [12]:
–reduced unproductive growth phase as a proportion of the total process time;
–more consistent product quality because conditions in the bioreactor are held stable;
–more extensive automation with improved process control.
From an economic point of view, perfusion cultures of animal cells should be operated at high perfusion rates [17]. However, the high cell concentrations achieved in such cases result in several technical constraints, such as oxygen transfer, CO2 removal, medium formulation, and, especially, cell retention efficiency.
Several different separation devices have been used for retaining cells in the bioreactor during perfusion cultures. They are usually based on centrifugal action (centrifuges, hydrocyclones), gravitational settling, filtration (spin-filters, tangential flow filters, dynamic filters), ultrasonic and dielectrophoretic separation. Besides the suitability for large-scale operation [18], other prerequisites for separation devices to be adequate for perfusion of mammalian cells are [16]:
–the retention efficiency of cells within the bioreactor should be high;
–stable, long-term operations should be possible;
–the device should be cleanable, sterilizable and reusable;
–it should maintain high culture viability, not damaging the cells by, for instance, mechanical shear stress, and should not induce changes in cell metabolism.
Cell retention devices can be placed internally in the bioreactor or externally, connected to it through a recirculation loop. Although internal devices are claimed to allow simple and safer operation [19], external devices permit that operation and scale-up parameters are completely decoupled from bioreactor operation and design. They also enable aseptic replacement in case of failure during long-term operation, allowing cultivation to continue. For instance, Trocha et al. [20] showed an example of a hybridoma perfusion cultivation over 1300 h, where several cell concentration declines could be observed throughout the cultivation, corresponding to failures in the retention device. When this happened, they switched to a second spare device, in order to allow the culture to continue. This is just one of various examples found in the literature reporting the lack of reliability of cell retention devices. In spite of the great effort that has been made in this field over the last ten years, this matter seems still not properly solved. In this context, the advantages and disadvantages of the different available devices will be discussed here.
2 Centrifugation
There are basically two families of centrifuges, the sedimenting centrifuges and the filtering centrifuges. The former uses centrifugal force to move the particles radially either outwards or inwards through a liquid, depending on whether
Cell Retention Devices for Suspended-Cell Perfusion Cultures |
135 |
they are heavier or lighter than the suspending liquid. The principle of separation of these centrifuges is, therefore, sedimentation in a centrifugal field and, as such, they will be seen in this item. The latter, also known as centrifugal filters, uses the centrifugal field to promote the necessary pressure to force the mother liquor through both the filter medium and the cake. Therefore, they are, in reality, filters.
Centrifuges can be very efficient when separating cells from culture media. Consequently, they can be used either in harvesting of mammalian cells [1,21–23] or, despite their mechanical complexity, as cell retention device in long-term perfusion systems (Table 3).
The degree of cell separation is an important parameter to be evaluated in perfusion systems. This can be done through the use of some concepts as cell separation efficiency, grade efficiency, and cut size. These concepts are applicable to any equipment whose performance remains constant if the operational conditions do not change. They are valid, therefore, for equipment such as sedimenting centrifuges, hydrocyclones, gravitational settlers, etc.
Eq. (1), known as Stokes’ law, gives the terminal settling velocity of a spherical particle settling in the laminar region (Stokes region) under the influence of a gravitational or centrifugal field.
vt |
= |
(ρ s − ρ ) bd2 |
(1) |
|
18η |
||||
|
|
|
where:
b = g for the gravitational field, b = w2R for centrifugal fields.
Eq. (1) is valid for a particle settling without the interference of other particles, i.e., for diluted systems. The particle settling velocity decreases as particle concentration increases. This phenomenon is known as hindered settling. Several equations can be found in the literature to account for this phenomenon, but a simple method to calculate the hindered settling velocity is to use Eq. (1) replacing the liquid density and viscosity by the apparent density and viscosity of the suspension [24].
Miller and Phillips [25] measured the terminal velocities due to gravity of some mammalian cells settling in a gradient of fetal calf serum in phosphate buffered saline at 4°C. Based on their experimental results, they proposed Eq. (2), which is a simplification of Eq. (1):
vt |
= |
r 2 |
(2) |
|
|||
|
4 |
|
where the terminal settling velocity vt is given in mm h–1 and r is the cell radius in µm.
Since first proposed, Eq. (2) has been used in some works [26, 27] for calculating terminal velocities of mammalian cells and even suggested in books [28]. Eq. (1) reduces to Eq. (2), for instance, for a density difference (Çs – Ç) of 0.05 g cm–3 and a medium viscosity (h) of 1.56 ¥ 10–3 Pa s (water viscosity at 4°C). Mammalian cells are usually cultivated at 37°C and the water viscosity at this
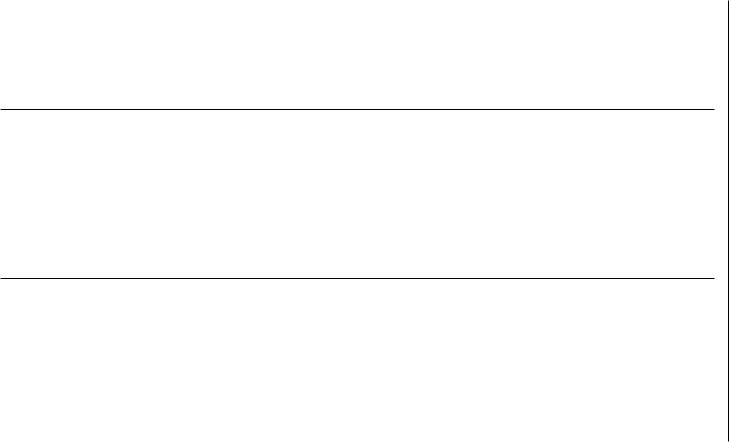
Table 3. Overview of reported perfusion processes employing centrifuges as cell retention device
Cell Line |
Product |
Reactor |
Max. |
Cultivation |
Centrifuge g-factora |
Feed Flow |
Max. Viable |
Separation |
Reference |
|||||
|
|
Volume |
Perfusion |
Time |
Type |
|
|
Rateb |
Cell Conc. |
Efficiency |
|
|||
|
|
(L) |
Rate (d–1) |
(d) |
|
|
|
(L min–1) |
(106 mL–1) |
(%) |
|
|
||
|
|
|
|
|
|
|
|
|
|
|
|
|
|
|
Hybridoma |
IgG |
6.0 |
5.5 |
12 |
disc-stack |
50 |
|
0.50 |
(C) |
20 |
|
100 |
|
40–42 |
HeLa |
– |
21.5 |
3.0 |
15 |
disc-stack |
50 |
|
0.72 |
(C) |
10 |
|
100 |
|
40–42 |
HeLa |
– |
21.5 |
3.8 |
27 |
disc-stack |
50 |
|
2.25 |
(C) |
10 |
|
100 |
Æ 95 |
40 |
CHO |
– |
100.0 |
10.1c |
16 |
disc-stack |
400 |
|
1.17 |
(C) |
>3 |
|
100 |
37 |
|
Hybridoma |
IgM |
1.3 |
0.5 |
18 |
centritech |
18 |
–49 |
0.04 |
(SC & I) |
3 |
–6 |
>99 |
|
44 |
Hybridoma |
IgG |
40 |
1.8 |
40 |
speciald |
50 |
|
0.2 (I) |
10 |
|
100 |
|
38–39 |
|
Hybridoma |
IgG |
10 |
2.0 |
37 |
speciald |
63 |
|
n.a. (C) |
12 |
|
n.a. |
|
35 |
|
Hybridoma |
IgG |
3 |
2.1 |
27 |
speciald |
100 |
|
n.a. (I) |
17 |
|
n.a. |
|
34 |
n.a.: Information not available. a g-factor = w 2 R/g.
b C: continuous operation, SC: semi-continuous, I: intermitent operation.
c Most of the supernatant was sent back to the reactor, in order to simulate the operation of a 1000 L reactor working at a 1 d–1 perfusion rate. d Rotor with a non-conventional design, containing 4 layers of spiral settling zones.
136
Medronho .A.R · Castilho .R.L

Cell Retention Devices for Suspended-Cell Perfusion Cultures |
137 |
temperature is 6.9 ¥ 10–4 Pa s. For the same density difference, and considering that the culture medium viscosity in perfusion cultures at 37°C is around 10% higher than the water viscosity, it is possible to deduce from Eq. (1) that the factor in Eq. (2) would change from 4 to 2. Under these conditions, Eq. (2) would then give a terminal settling velocity around half of the correct value. Therefore, since viscosity is a strong function of temperature, the use of Eq. (2) should be avoided. Instead, Eq. (1) should be employed.
The main streams of a separator are usually three: the feed stream containing the cells to be separated, the underflow containing the separated cells, and the overflow containing the cells that the separator could not capture. The cell separation efficiency (or retention efficiency) E is defined by Eq. (3), and gives the fraction of cells recovered in the underflow.
E = |
Qu X u |
(3) |
QX
The fraction of fluid discharged in the underflow is called flow ratio Rf . Separators can operate with or without a fluid flow rate in the underflow. For instance, tubular centrifuges operate usually with Rf = 0, and scroll type centrifuges and hydrocyclones with Rf > 0. A flow ratio equal to Rf means that this fraction of feed fluid is leaving the separator through the underflow. Since the fluid carries solid particles with it, some particles will be discharged into the underflow not due to the centrifugal action of the separator but due to entrainment. In spite of some controversy regarding hydrocyclones [29–31], this bypass is normally assumed to be equal to the flow ratio [24, 32]. Therefore, Rf is the minimal efficiency at which a separator will operate even if no centrifugal action takes place. For the conditions usually found in mammalian cell cultures:
R f |
Qu |
(4) |
|
Q |
|||
|
|
The reduced separation efficiency E¢ (Eq. 5), also called centrifugal efficiency, is used for separators with Rf > 0, and gives the separation efficiency taking into account only those particles that will be separated or not due to the centrifugal field intensity. Hence, E¢ does not consider the particles that are separated due to entrainment in the overflow stream.
E' = |
E − |
R f |
(5) |
|
1− |
Rf |
|||
|
|
Based on Eqs. (3), (4) and (5), and on a material balance on the separator:
E' = 1− |
X o |
(6) |
X
The same concepts on separation efficiency expressed by Eqs. (2) and (4) can be applied to a given size of cell, generating curves of efficiency as a function of cell size [33].
138 |
L.R. Castilho · R.A. Medronho |
Mammalian cells may be affected by the relatively high shear stresses present in separation under centrifugal fields. Hamamoto et al. [34] found that exposing hybridoma cells to a centrifugal acceleration intensity of 100 g twice a day for 30 minutes did not affect the cell growth rate. However, accelerations of 500 g caused some depression on cell growth. Tokashiki et al. [35], also working with hybridoma cells, found no effect on either cell growth or MAb specific productivity for accelerations varying from 100 g to 500 g twice a day for 10 minutes. However, in a similar experiment using another hybridoma cell line, Tokashiki and Yokoyama [36] found a 50% decrease in the antibody production at 500 g, although no effect was observed at 200 g. The authors concluded, then, that the influence of centrifugal fields on cell proliferation and product formation is a function of the cell type. Nevertheless, higher stressing conditions have been imposed on cells, without noticeable effects on cell growth, viability or product formation [37].
Tokashiki et al. [35] showed that it was possible to use continuous centrifugation for cell retention in perfusion cultures. Their results for viable cell concentration and IgG specific productivity were equivalent to the results obtained with a centrifuge operating in a intermittent fashion or with a gravitational settler [34]. In another work, the same research group [38, 39] proposed a novel centrifuge with multiple settling zones to separate cells from culture medium. They were able to run a perfusion system for 40 days using the new centrifuge in an intermittent fashion. A disadvantage of this equipment is the necessity of introducing a liquid carrier (e.g. perfluorocarbon) to wash the settled cells from the centrifuge back to the reactor. This liquid carrier has to remain inside the reactor during the whole culture time.
Among the conventional industrial centrifuges, only the disc-stack type has been studied as an option of cell retention device for perfusion bioreactors. Jäger [40–42] used a continuous disc-stack centrifuge (Westfalia CFA-01) to conduct perfusion cultures of mammalian cells. When cultivating hybridoma cells in a 6 L reactor, the author used a 0.5 L min–1 feed flow rate and, to avoid cell settling in the pipes, he worked with a flow ratio of 95.4%. At these feed rates, cells were passing through the centrifuge every 12 min and the perfusion was kept for 6 days. Even under these extremely hard conditions, Jäger did not observe any significant effect on viability and only a slight effect on cell growth (population doubling time changed from 25.3 h in batch to 27.7 h in perfusion). Even better results were obtained when cultivating HeLa cells in a 21.5 L bioreactor. In this case, the viabilities were kept for most of the perfusion time (10 days) over 95% and the doubling time was slightly better when compared with the batch period (26.3 h vs 28.7 h). The feed flow rate in this experiment was 0.72 L min–1 and the flow ratio was 93.7%. A longer perfusion run (25 days) was then accomplished by the author [40] using higher feed flow rate (2.25 L min–1) and flow ratio (97.5%). In this case, the author noticed that one of the 18 pouches that direct the separated cells to the centrifuge underflow was clogged.
Different results regarding clogging were obtained by Björling et al. [37] when using the same centrifuge as Jäger [40–42]. These authors evaluated the separation efficiency of CHO cells from the culture medium using rotor speeds