
Orbital Interaction Theory of Organic Chemistry
.pdf
EFFECT OF X: SUBSTITUENTS |
99 |
…a† |
…b† |
…c† |
Figure 6.1. Summary of the most common electrophilic addition reactions of ole®ns. In each case, the ole®n reacts as a Lewis base. All reactions are regioselective. The overall stereochemistry is (a) stereospeci®c anti; (b) stereospeci®c syn; (c) not stereospeci®c, in general.
de®ned by Fleming, namely X: (p electron donors), Z (p electron acceptors), and ``C'' (conjugating) [7]. An interaction diagram showing the interaction of a CÐC p bond with each type of substituent is shown in Figure 6.2. We note the e¨ect of the substituent on the energy and polarization of the p bond at its original site.
EFFECT OF X: SUBSTITUENTS
As shown in Figure 6.2a, an X: substituent, which has a p orbital or other suitable doubly occupied orbital which will interact with the p bond, raises the energy of the HOMO and LUMO, thus rendering the ole®n more reactive as a Lewis base. Of course, the electrons of the HOMO are also delocalized onto X. The probability of attack by an

100 REACTIONS AND PROPERTIES OF p BONDS
…a† |
…b† |
…c† |
Figure 6.2. Interaction of CÐC with (a) X:; (b) Z; (c) C-type substituents.
electrophile will be governed by the magnitude of the coe½cient at the particular atomic position. Polarization of the HOMO away from the point of attachment of the X substituent directs electrophilic attack to that carbon. Attack may also be directed to X itself in certain cases, although this is usually reversible and may have no net consequences. The X-substituted ole®n p system is isoelectronic to that of the allyl anion (Figure 5.2). The polarization and energy of the p bond can be deduced by averaging the HOMOs and HOMO energies of the ole®n and allyl anion:
The X: substituents are ÐNR1R2, ÐOR, ÐSR, ÐF, ÐCl, ÐBr, ÐI, or ÐCH3 (or any alkyl). The R's may be H or alkyl, or aryl, or even acyl. Thus,
CH3CHbbCH2 ‡ HaaaOSO2OH ! CH3C‡HaaaCH3 ‡ ÿOSO2OH
…not CH3CH2 aaaC‡H2†
As a special case, consider the aldol reaction
CH2 bbCHOÿ ‡ CH3CHO ! CH3C…Oÿ†HaaaCH2CHO
The enolate anion may be considered as an alkene with a very good powerful X:-type substituent, the alkoxide oxygen. The HOMO of the p bond is strongly raised in energy
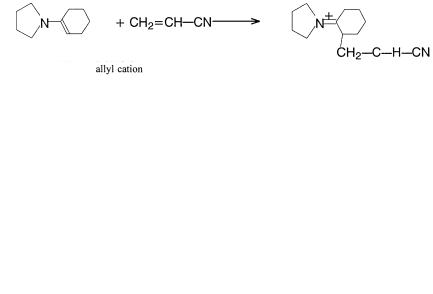
EFFECT OF ``C'' SUBSTITUENTS |
101 |
and polarized away from the alkoxy substituent. The weak electrophile, the carbonyl of acetaldehyde, adds at the distal C atom.
EFFECT OF Z SUBSTITUENTS
As shown in Figure 6.2b, a Z substituent, which has a p or p orbital or other suitable empty low-lying orbital which will interact with the p bond, lowers the energy of the HOMO and LUMO, thus rendering the ole®n less reactive as a Lewis base but more reactive as a Lewis acid. The electrons of the HOMO are also delocalized onto Z. The probability of attack by an electrophile will be governed by the magnitude of the coe½cient at the particular atomic position. Polarization of the LUMO away from the point of attachment of the Z substituent directs nucleophilic attack to that carbon. Attack may also be directed to Z itself in certain cases, and this may be irreversible, providing an alternate pathway for the reaction. The Z-substituted ole®n p system has some characteristics of an allyl cation (Figure 5.2). The polarization and energy of the p orbital can be deduced by averaging the LUMOs and LUMO energies of the ole®n and allyl cation:
The Z substituents are ÐCOR, ÐCN, ÐSOR, ÐSO2R, ÐNO, and ÐNO2. The R's may be H, alkyl, aryl, or even X: substituents. Thus, the Michael addition is the best example:
CH2 bbCHaaaCO2CH2CH3 ‡ CHÿ…CO2CH2CH3†2 ! CH2 aaaCÿHaaaCO2CH2CH3
CH…CO2CH2CH3†2
Consider also
EFFECT OF ``C'' SUBSTITUENTS
As shown in Figure 6.2c, a ``C'' substituent, which has evenly spaced p and p orbitals, raises the energy of the HOMO and lowers the LUMO, thus rendering the ole®n more reactive as both a Lewis base and a Lewis acid. The electrons of the HOMO and LUMO are also delocalized onto ``C.'' The probability of attack by an electrophile or nucleophile will be governed by the magnitude of the coe½cient at the particular atomic position. Polarization of both the HOMO and LUMO away from the point of attachment of the ``C'' substituent directs attack to that carbon. Attack may also be directed to ``C''
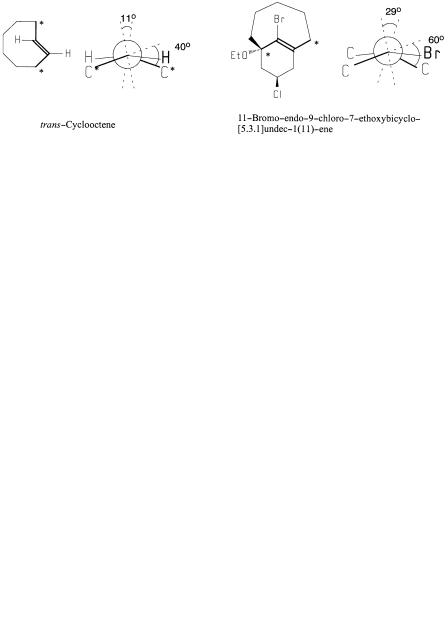
102 REACTIONS AND PROPERTIES OF p BONDS
itself in certain cases. The ``C'' substituted ole®n p system is assumed to be butadienelike (Figure 5.2), and hence the polarization of the p and p orbitals:
The ``C'' substituents are alkenyl, alkynyl, or aryl groups.
EFFECT OF DISTORTION OF MOLECULAR SKELETON
Ole®ns may be synthesized in which the p bond must be superimposed on a s framework which deviates signi®cantly from the ideal geometry, namely coplanarity with a two sp2 hybridized carbon atoms, or in the case of alkynes, collinearity. Twisting of the two ends of the double bond relative to each other has the consequence of reducing the p overlap and hence the resonance integral is less than jbCCj. The higher HOMO means that the twisted ole®n is more susceptible to electrophilic attack, the lower LUMO implies an increased susceptibility to nucleophilic attack, and the smaller HOMO±LUMO gap suggests a bathochromic shift for the pp electronic transition [115]. The increased susceptibility of twisted (strained) alkenes toward electrophilic attack has been demonstrated experimentally for MCPBA (meta-chloroperbenzoic acid) epoxidation [116] of a variety of strained alkenes. The rate enhancement was attributed to relief of strain in the transition state, but a correlation was noted with ionization potential, and hence the energy of the HOMO. Conjugated alkenes which have high HOMO energies also were less reactive than expected on the basis of the correlation with twisted monoalkenes. In orbital interaction terms, the reduced reactivity of conjugated systems is attributed to the smaller orbital coe½cients and hence lower intrinsic interaction matrix elements [see equation (3.47)].
In fact, it is di½cult to e¨ect a major perturbation to the ole®n p system by twisting. For instance, the s framework of trans-cyclooctene is twisted by 40 out of planarity, but pyramidalization at each end is such as to reduce the twist of the p orbitals to only about 11 [117]:
The ionization potential, 8.69 eV, is lower than in the case of cis-cyclooctene (8.98 eV) or cyclohexene (9.12 eV), as expected. The highly strained anti-Bredt ole®n, 11-bromo- endo-9-chloro-7-ethoxybicyclo[5.3.1]undec-1(11)ene has been synthesized and its struc-
p BONDS TO SILICON, PHOSPHORUS, AND SULFUR |
103 |
ture determined by X-ray di¨raction [118]. The twist in the s framework is approximately 60 . As in the case of trans-cyclooctene, both ends of the p bond are pyramidally distorted in such a way as to reduce the twist angle of the p orbitals to 29 .
ALKYNES
At the level of simple orbital interaction theory, alkynes di¨er from alkenes in only two respects. First, the carbon atoms are dicoordinated, with the consequence that the p orbitals are at higher energy than aC. Second, the shorter CÐC separation implies that the intrinsic interaction will be larger than bCC. The level of the degenerate p MOs is expected to be about the same as in alkenes but the p MOs will be higher in energy. Additional factors such as increased coulombic repulsion of the two electrons in each p MO (due to the shorter separation) may further destabilize the p MOs. In fact, the reactivity of alkynes toward electrophilic attack is rather similar to that of alkenes when the electrophile would be expected to form an acyclic intermediate (e.g., HX) but slower when a cyclic intermediate is formed (e.g., Br2) [114]. Addition of nucleophilic free radicals to alkynes proceeds more slowly than to alkenes, an observation consistent with the expected higher p MO of the former. However, addition of nucleophiles is faster in general than to alkenes [119, pp. 670±62]. Since strong charged nucleophiles are always associated with a metallic counterion, the Lewis acidity of the cation toward the second ``nonreacting'' p MO may be responsible for the increased reactivity.
p BONDS TO AND BETWEEN HIGHER ROW ELEMENTS
The principles discussed in Chapter 4 for the case of s bonds to higher row elements apply with a vengeance in the case of p bonds. Thus p bond orbital energies and polarizations are determined not so much by n p orbital energy di¨erences as by the smaller intrinsic interaction matrix elements, bCX or bXY, for p orbitals with principal quantum numbers n > 2. Smaller bCX or bXY values are associated with decreased HOMO± LUMO gaps, decreased LUMO energies, increased HOMO energies, and higher polarizations, all of which dictate substantially decreased thermodynamic and kinetic stabilities. Indeed, besides the 2p±2p p bonds discussed above, the only other combination in which it still makes sense to consider p±p p bonds is the 2p±3p case, that is, bonds of the type CÐSi, CÐP, and CÐS, the third-row analogues of alkenes, imines, and carbonyls. Note that orbital nodal characteristics and size do favor p bonds of the 2p±n d type, especially 2p±3d. The case of organometallic bonding is treated in Chapter 13.
p BONDS TO SILICON, PHOSPHORUS, AND SULFUR
The SHMO description of p bonds CÐC, CÐSi (silaethenes), and SiÐSi (disilenes) are compared in Figure 6.3 using parameters taken from Table 5.1. According to the parametrization of Van Catledge [105], C and Si have the same value of hX (i.e., 0.0), but this may not be correct since Si is substantially less electronegative than C (Table A.2). The intrinsic interaction matrix elements decrease in the following series: CÐC, ÿ1:00jbj; CÐSi, ÿ0:75jbj; SiÐSi, ˆ ÿ0:64jbj. Thus, the p bond energy decreases accordingly, but signi®cant bond polarization is not predicted. The decreased HOMO±LUMO gap,

104 REACTIONS AND PROPERTIES OF p BONDS
…a† |
…b† |
…c† |
Figure 6.3. SHMO description of p bonds: (a) CÐC; (b) CÐSi; (c) SiÐSi.
decreased LUMO energies, and increased HOMO energies of the CÐSi and SiÐSi species indicate substantially decreased thermodynamic and kinetic stabilities. Indeed, these species are in general not isolable but have been postulated as reactive intermediates [120, 121]. Silaethene, CH2SiH2, has been isolated in an argon matrix [122]. Its p bond energy has been determined experimentally, 142 G 17 kJ/mol [123], and theoretically, 132 kJ/mol [124], to be substantially lower than the p bond energy of ethylene, 269 kJ/mol [125]. The highly hindered tetramesityldisilene has been isolated [126], but not the parent, SiH2SiH2, whose p bond energy has been calculated to be only 97 kJ/mol [124]. In both silaethenes and disilenes, the silicon atom is not planar, but fairly strongly pyramidal [124].
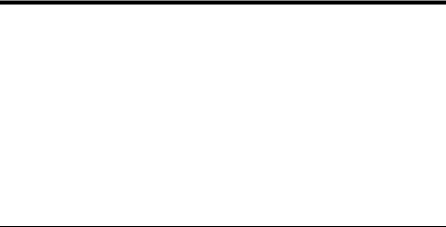
Orbital Interaction Theory of Organic Chemistry, Second Edition. Arvi Rauk Copyright ( 2001 John Wiley & Sons, Inc.
ISBNs: 0-471-35833-9 (Hardback); 0-471-22041-8 (Electronic)
CHAPTER 7
REACTIVE INTERMEDIATES
REACTIVE INTERMEDIATES [CH3]B, [CH3]C, [CH3]., AND [:CH2]
The major carbon centered reaction intermediates in multistep reactions are carbocations (carbenium ions), carbanions, free radicals, and carbenes. Formation of most of these from common reactants is an endothermic process and is often rate determining. By the Hammond principle, the transition state for such a process should resemble the reactive intermediate. Thus, although it is usually di½cult to assess the bonding in transition states, factors which a¨ect the structure and stability of reactive intermediates will also be operative to a parallel extent in transition states. We examine the e¨ect of substituents of the three kinds discussed above on the four di¨erent reactive intermediates, taking as our reference the parent systems [CH3]‡, [CH3]ÿ, [CH3]., and [:CH2].
Carbocations
Figure 7.1 shows the interaction diagrams relevant to a carbocation substituted by X:, Z, and ``C'' substituents:
.A carbocation is strongly stabilized by an X: substituent (Figure 7.1a) through a p-type interaction which also involves partial delocalization of the nonbonded electron pair of X to the formally electron-de®cient center. At the same time, the LUMO is elevated, reducing the reactivity of the electron-de®cient center toward attack by nucleophiles. The e¨ects of substitution are cumulative. Thus, the more X:-type substituents there are, the more thermodynamically stable is the cation and the less reactive it is as a Lewis acid. As an extreme example, guanidinium ion, which may be written as [C(NH2)3]‡, is stable in water. Species of the type [ÐC(OR)2]‡ are common intermediates in acyl hydrolysis reactions. Even cations stabilized by ¯uorine have been reported and recently studied theoretically [127].
105
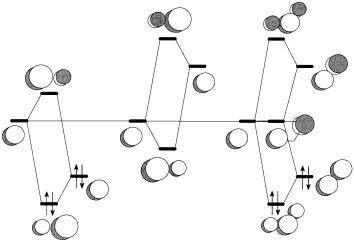
106 REACTIVE INTERMEDIATES
…a† |
…b† |
…c† |
Figure 7.1. Carbocationic center interacting with (a) an X: substituent; (b) a Z substituent; (c) a ``C'' substituent.
The ®lled bond orbitals of adjacent alkyl groups may donate electrons by p-type overlap (see Figures 3.19 and 3.20 in Chapter 3) to an adjacent carbocationic center. Thus, an alkyl group may be considered to be an X-type substituent. The highest combination of the CÐH bonding orbitals of a methyl group has a p donor capability intermediate to that of the nonbonded orbitals of O and F. The donor abilities of s bonds were discussed in Chapter 4.
.A carbocation is only weakly stabilized by a Z substituent (Figure 7.1b) through a p-type interaction with the p bond of the Z group (not shown in Figure 7.1b). The interaction is weak because the p bond of a Z substituent is very low in energy and polarized away from the cationic center. The dominant interaction is with the LUMO of Z, which does not add to thermodynamic stabilization but greatly enhances the Lewis acidity of the cation, increasing the reactivity of the electron-de®cient center toward attack by nucleophiles. The Z-substituted cations are being increasingly reported as intermediates in solvolysis reactions [128].
.A carbocation is strongly stabilized by a ``C'' substituent (Figure 7.1c) through p- type interactions which involve substantial delocalization into the substituent. The LUMO energy is relatively unchanged, but the reactivity of the electron-de®cient center toward attack by nucleophiles is reduced because the orbital coe½cients are smaller. Allyl and benzyl carbocations are prototypical of ``C''-substituted carbocations. The e¨ects of substitution are cumulative. Thus, the more ``C''-type substituents there are, the more thermodynamically stable is the cation and the less reactive it is as a Lewis acid. A prime example is triphenyl carbocation.
Intermolecular Reactions of Carbocations
Carbocations are strong Lewis acids which occur as intermediates in reactions following the SN1 (Chapter 9) or E1 (Chapter 10) mechanistic routes. The most obvious and

REACTIVE INTERMEDIATES [CH3]‡, [CH3]ÿ, [CH3]., AND [:CH2] |
107 |
common reaction is recombination with a nucleophile (a Lewis base) to form a s bond:
If the nucleophilic site (HOMO) involves a nonbonded pair of electrons (path a), a stable covalently bonded complex will form. If the HOMO is a s bond, direct reaction is unlikely unless the bond is high in energy and sterically exposed, as in a three-membered ring, but if the bond is to H, hydride abstraction may occur (path b, steps 1 and 2) or a hydride bridge may form (path b, step 1). The last two possibilities are discussed further in Chapter 10. If the HOMO is a p bond, a ``p complex'' may result (path c, step 1), or, more commonly, donation of the p electrons results in the formation of a s bond at the end where the p electron density was higher, the other end becoming Lewis acidic in the process (path c, steps 1 and 2). The e¨ects of substituents on ole®n reactivity were discussed in Chapter 6.
Intramolecular Reactions of Carbocations
Intramolecular reactions of carbocations are shown in the following scheme:
The combination of steps 1 and 2 corresponds to a 1,2 hydride shift (R ˆ H) or a Wagner± Meerwein rearrangement (R ˆ alkyl). The intermediate bridged nonclassical structure
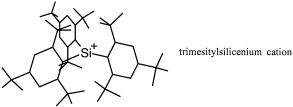
108 REACTIVE INTERMEDIATES
reached after step 1 may correspond to a transition state for the rearrangement, or the stable form of the carbocation. If R ˆ H, the bridged form is known to be stable only in the case of ethyl cation, where there are no substituents on either carbon atom (other than H). In other cases, stable bridged forms are obtained if the R group has a relatively loosely bound pair of electrons in a p- or p-type orbital for back donation into the p - like orbital of the CÐC fragment, as in the case of R ˆ halide (e.g., bromonium ion), R ˆ aryl (e.g., ethylbenzenium ion [129]), or R ˆ vinyl (cyclopropylcarbinyl cation). More or less symmetrical bridging by R ˆ alkyl is not likely but has been shown to be the case in the 2-norbornyl cation [98, 130]. Depending on substituents in R, elimination of R‡ may occur (step 3). Each of the cationic species may react intermolecularly, as shown in the previous scheme at sites labeled A. If R ˆ H, the hydrogen end of the s bond in the two ``classical'' structures is also liable to attack by nucleophile (the E1 mechanism, Chapter 10). Of course, step 3 would not occur in this case.
Silyl Cations
The silicon analogs of carbocations have been reported a number of times [131], but there is only one con®rmed ``sighting'' to date, the highly hindered trimesitylsilicenium ion as its tetrakis(penta¯uorophenyl)borate salt [132]:
From the point of view of kinetic or thermodynamic stability of silyl cations, what can be deduced from orbital interaction theory? Silicon is less electronegative than carbon (Table A.2), so on the face of it, the empty 3p orbital energy should be higher than the energy of the 2p orbital of C. However, this is probably not true in the case of stabilized carbocations since strong 2p±2p p-type stabilizing interactions will raise the LUMO well above jaj and result in extensive delocalization (lower the coe½cient at C). On the other hand, the much weaker 3p±2p p-type interactions will have little e¨ect in raising or delocalizing the LUMO of silyl cations, which will remain as very powerful Lewis acids, coordinating with solvent and possibly undergoing intramolecular group migrations to give the relatively much stronger s bonds to Si. Thus, silyl cations are thermodynamically stable but kinetically very reactive.
Carbanions
Except for the most highly stabilized carbanions, carbanion chemistry in solution is always complicated by the presence of the counterion, usually a metal, which is a Lewis acid and almost invariably is involved in the course of the reaction. Relative stabilities of carbanions in solution are di½cult to establish for the same reason. In recent years, much information has been gathered about carbanion stabilities, structures, and reactiv-