
- •LETTERS
- •Cocktail party at the beginning of the universe
- •Domingo de Soto, early dynamics theorist
- •Making partners of universities and corporations
- •Correcting the record of manmade VLF radiation
- •Direct imaging reveals exoplanets in orbital motion
- •Pressure unites two regimes of fluid breakup
- •Nuclear weapons at a crossroads as Obama enters office
- •Applying Title IX to university science departments
- •DOE invites partners in green technology
- •LBNL director Chu to head DOE
- •NASA sells technology rights to highest bidder
- •Scientists entertain Hollywood queries
- •Europe sets astronomy path
- •US, China settle on nuclear terms
- •Solving quantum field theories via curved spacetimes
- •Panofsky agonistes: The 1950 loyalty oath at Berkeley
- •OPINION
- •BOOKS
- •William and Lawrence Bragg, Father and Son
- •Energy in Nature and Society
- •The Bethe–Peierls Correspondence
- •NEW PRODUCTS
- •OBITUARIES
- •Theodore Eugene Madey
- •Julius Erich Wess
- •The versatility of nanoscale mechanical resonators
- •Biomimetic ceramics
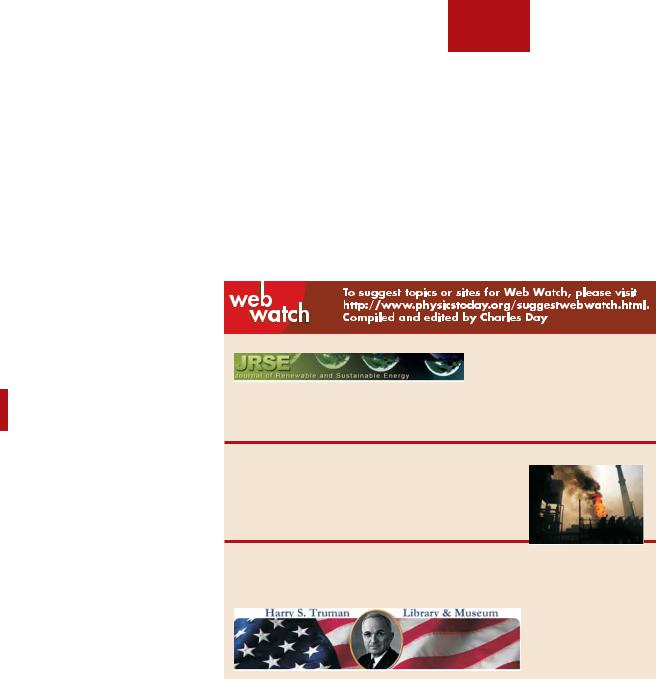
Extremely Large Telescope (EELT) and the Square Kilometre Array radio telescope top the priority list for groundbased facilities that exceed €400 million. The top picks in the medium scale (€50 million– €400 million) are the European Solar Telescope, the Cherenkov Telescope Array, and then KM3NeT, an underwater neutrino detector—the last two were priorities in the recent astroparticle roadmap (see PHYSICS TODAY, November 2008, page 25). The two highest-ranked space missions are the Laser Interferometer Space Antenna, a gravitational wave observatory, and the International X-ray Observatory. The full report is available at http://www.astronet-eu.org.
As a follow-up, Astronet has commissioned a review of Europe’s 2- to 4-meter optical telescopes. The “heterogeneous mix of national and common instruments, equipped and operated without overall coordination,” creates a situation that “impedes effective ground-based support of space missions,” says Johannes Andersen, Astronet board chair and director of the Nordic Optical Telescope on La Palma. A review of Europe’s millimeter–submillimeter and radio telescopes is also planned, as is one focusing on optimizing exploitation of 8- to 10-meter-class optical telescopes in the era of the EELT.
One goal of the roadmap, says Andersen, “is to define a common European strategy on future global-scale projects. We hope this will be helpful input to the new decadal survey in the US.” Implementing the roadmap will require an increase of roughly 20% in current astronomy spending, which totals about €2 billion a year.
Toni Feder
US, China settle on nuclear terms
How does one define “assassin’s mace”? The expression proved to be one of the most difficult of the 1000 or so terms that a US–China team of nuclear experts had to wrestle with as they assembled a common glossary that can be used in bilateral and international discussions involving nuclear security issues. The two-year project, sponsored by the US National Academy of Sciences’ Committee on International Security and Arms Control (CISAC) and the Chinese Scientists Group on Arms Control of the Chinese People’s Association for Peace and Disarmament, released its product in November; publication had been delayed for two months by the disastrous May earth-
26 January 2009 Physics Today
quake in Sichuan Province.
Most of the glossary entries are simply matched to their equivalents in the other language. But some terms required more nuanced translations. “New thinking,” for example, continues in the Chinese policy lexicon to denote the geopolitical philosophy advanced by Soviet president Mikhail Gorbachev two decades ago. “Selfdefensive nuclear strategy” is China’s official description of its no-first-use policy for nuclear weapons. Even “nuclear weapon” required four definitions, including one used by the North Atlantic Treaty Organization and another by the Russian government. On the other hand, both sides judged the terms “second-generation nuclear weapon” and “theory of low-intensity conflict” to be clear enough to stand alone. “The process was enormously useful,” declared CISAC chair Raymond Jeanloz of the University of California, Berkeley. “The Chinese discussions provided a richer understanding of the ambiguities and implications” of translations.
The Chinese side initially resisted inclusion of assassin’s mace, or shashou jian, dismissing it as US jargon, said Ming-Shih Lu, chair of the CISAC panel that produced the glossary. In US geopolitical parlance, the term describes a Chinese strategy to overcome a superior adversary with a quick technological knockout blow, particularly
in connection with a possible US intervention in a conflict between Taiwan and the mainland. When informed that former Chinese president Jiang Zemin had once used the term in a speech, the Chinese agreed to include it. Still, something may have been lost in the translation: Assassin’s mace is defined as “a type of metal weapon” that is thrown unexpectedly at an adversary and as “a metaphor for an adept ability or unique skill used at a critical moment.”
The glossary is available online at http://www7.nationalacademies.org/ cisac/Glossary_CISAC.html.
David Kramer
Oppenheimer TV docudrama. On Monday,
26 January, the Public
Broadcasting System will premiere The Trials of J. Robert Oppenheimer, a two-hour program centered around a dramatic re-creation of the 1954 US government hearings that confirmed the 1953 revocation of Oppenheimer’s security clearance. During World War II, the theoretical physicist had been the head scientist of the Manhattan Project, which developed the atomic bomb. And after the war, he had served the government as a key adviser on nuclear-weapons issues. The dramatization of the hearings, with actor David Strathairn portraying the beleaguered Oppenheimer, is interspersed with commentaries by historians and scientists,
http://jrse.aip.org
The Journal of Renewable and Sustainable Energy is the newest
publication from the American Institute of Physics. Published online but not in print, JRSE covers all aspects of the generation, conversion, and distribution of green energy. The journal’s peer-reviewed papers are complemented by a blog, news picks, and interviews with scientists and policymakers.
http://www.foreignpolicy.com/story/cms.php?story_id=4555
Writing in the journal Foreign Policy, Richard Muller has drawn up The List: Five Physics Lessons for Obama. The succinct lessons cover terrorism, energy, nuclear energy, space, and global warming. Muller is a physicist at the University of California, Berkeley.
http://www.trumanlibrary.org/whistlestop/ study_collections/bomb/large
The Harry S. Truman Library and Museum has been steadily scanning its trove of presidential records and making them available online. The collection about Tru-
man’s decision to drop atomic bombs on Hiroshima and Nagasaki now includes more than 70 items.
www.physicstoday.org
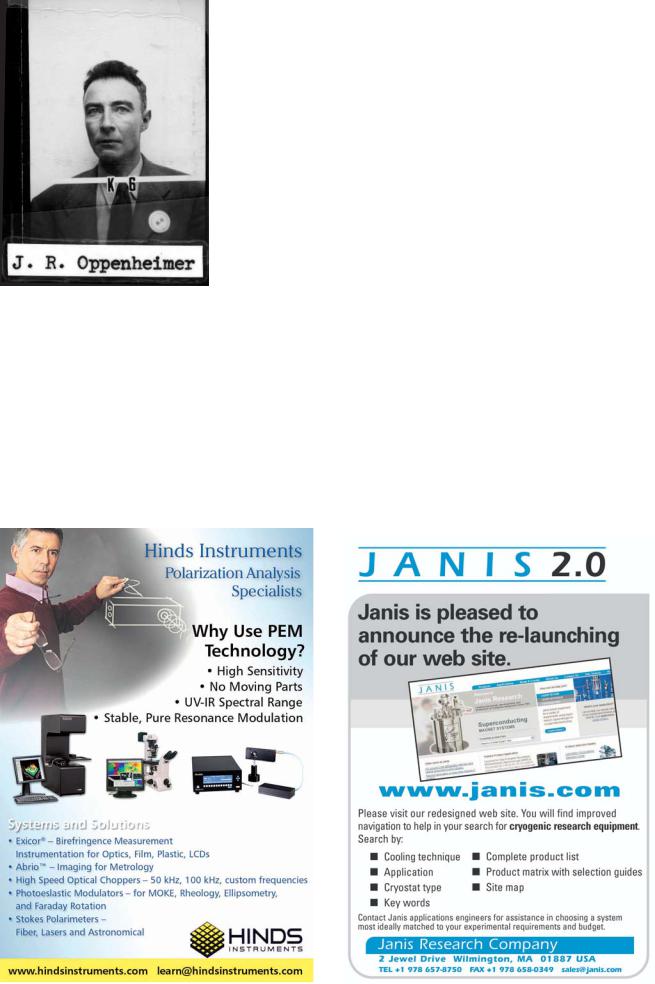
LOS ALAMOS NATIONAL LABORATORY
photos from Oppenheimer’s life, and historic film and TV footage. The program, part of the PBS American Experience series, was written, produced, and directed by David Grubin. BMS
Dubai forum. “The Biology of Busi- ness—Learning from Nature to Understand Business” was the title of Geoffrey West’s talk last November at the fifth annual Leaders in Dubai Business Forum. West, a physicist and the president of the Santa Fe Institute, was the first scientist to be invited to participate in the forum.
West was asked to attend, according
to a Leaders in Dubai spokesperson, because his “scientific theories are directly related to the business world” and because the forum last year wanted to “provoke new ways of thinking and see a new viewpoint on why businesses and organizations behave the way they do.” What caught the organizers’ attention, says West, “was work I’ve done showing why the pace of life increases, and why we have to be innovating at an accelerated rate.”
In Dubai, West shared the stage with the likes of Mohamed ElBaradei, director general of the International Atomic Energy Agency; Rudolph Giuliani, former mayor of New York City; and James Wolfensohn, former World Bank president. The forum was attended by some 1500 business leaders from across the Middle East. TF
Preventing an attack. A terrorist attack using a weapon of mass destruction is likely to occur somewhere in the world before the end of 2013, unless concerted and urgent international actions are taken, a congressionally chartered commission has warned. Although a bioterror attack is more likely than one involving a nuclear explosive, the Commission on the Prevention of Weapons of Mass Destruction Proliferation and Terrorism said in its 2 De-
cember report that the nuclear threat continues to increase as North Korea and Iran gain nuclear weapons capabilities. In addition to recommending reinvigoration of the nonproliferation agenda, the bipartisan commission, chaired by former senators Bob Graham (D-FL) and Jim Talent (R-MO), called for a “radical revamp” of US policy toward Pakistan, which it described as “the crossroads of proliferation and terrorism.” The US must use diplomatic, military, and economic means to work with Pakistan and other governments in the region to eliminate terrorist safe havens, it said.
Among the commission’s recommendations are the establishment of an international nuclear fuel bank to supply reactor fuel to, and take back spent fuel from, nations that pledge to not acquire their own fuel production capacity, and the provision of additional authorities and more funding for the International Atomic Energy Agency. And, in what panel member Henry Sokolski of the Nonproliferation Policy Education Center described as “a radical recommendation,” the commission said that US subsidies for the promotion of nuclear power in other nations should be discouraged.
The commission’s report is available at http://www.preventwmd.gov. DK
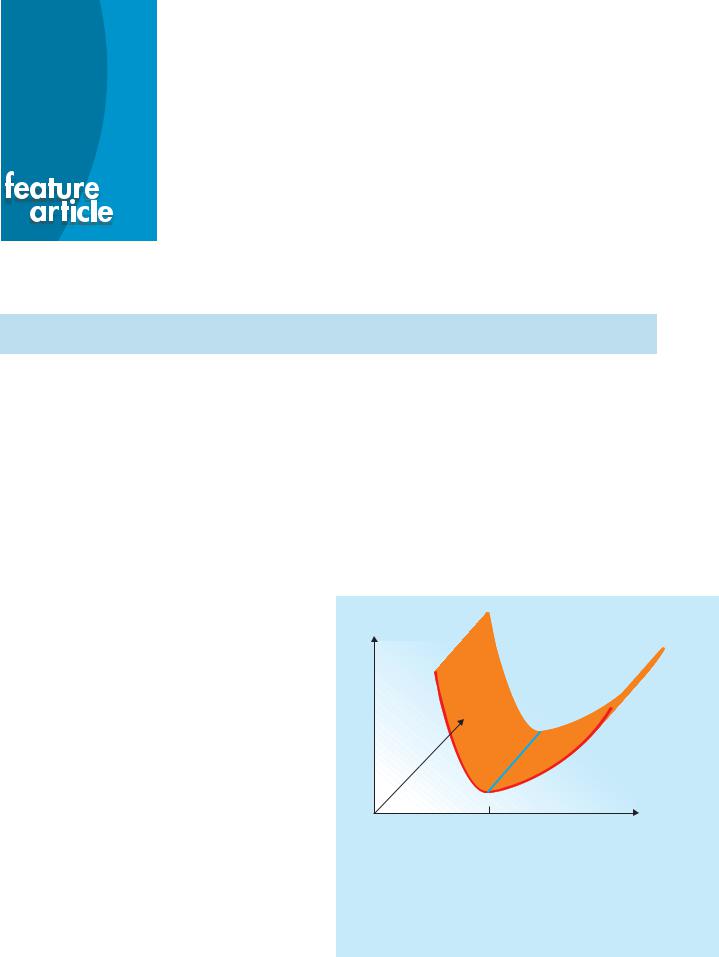
Solving quantum field theories via curved spacetimes
Igor R. Klebanov and Juan M. Maldacena
Strongly interacting quantum field theories are notoriously difficult to work with, but new information about some of them is emerging from their surprising correspondence with gravitational theories.
Igor Klebanov is a professor of physics and associate director of the center for theoretical science at Princeton University in Princeton, New Jersey. Juan Maldacena is a professor at the Institute for Advanced Study in Princeton.
Eleven years ago several theorists proposed a remarkable correspondence between two seemingly different kinds of theories.1 It is often called a duality because it is an equivalence between two different, “dual” descriptions of the same physics. On one side of the duality are certain quantum field theories (QFTs)—for example, Yang–Mills gauge theories similar to those in the standard model of particle physics. Such theories describe interacting particles moving in a flat d-dimensional spacetime. On the other side are theories that include gravity, like Albert Einstein’s general relativity or its string-theoretic generalizations. The gravitational theories are defined in a higher-dimensional spacetime containing at least the d dimensions of the particle theory plus one extra dimension of infinite extent. They often include a number of finite dimensions—in the form of a sphere, for example. Depending on the context, the correspondence is known as a gauge/gravity duality, gauge/string duality, or AdS/CFT (anti–de Sitter/conformal field theory) correspondence. Do not despair if the terminology is unclear. In this article we will attempt to describe in simple terms what the duality is and how and why it is useful for studying a variety of problems.
The gravitational theory involves a dynamic spacetime fluctuating around a special curved background: The gravitational potential energy rises sharply as the coordinate y of the extra dimension approaches infinity. To motivate the correspondence, we consider a class of curved spacetimes for which the gravitational potential energy has an absolute minimum at y = 0, as in figure 1. The correspondence asserts that the dynamics of the d + 1–dimensional gravitational theory is encoded in a d-dimensional QFT. That should not be too surprising: In the presence of a potential energy rising sharply along a particular spatial direction, one expects the lowenergy dynamics of a theory to become effectively confined to the remaining dimensions. The gauge/string duality, however, goes beyond that simple intuition because even motion along the extra dimension is reproduced by the corresponding d-dimensional QFT.
The duality is particularly useful for studying certain QFTs that are very strongly coupled because then the corresponding gravitational theory is defined in a weakly curved spacetime and readily analyzed using the methods of general relativity. On the other hand, the fact that weak curvature cor-
responds to strong coupling makes the duality hard to prove because it is difficult to do reliable computations in a strongly coupled field theory. Nonetheless, theorists have accumulated a great deal of evidence in its favor.
If one simply assumes that the duality is true, then it provides a wealth of new information about strongly coupled field theory. The collection of theories that can be solved using the duality is still rather limited; it does not contain any theory that describes a known physical system. Yet the theories that can be solved capture some essential features of theories realized in nature. One of the best-studied examples is a cousin of quantum chromodynamics, the field theory describing strong nuclear forces. The cousin, though, includes supersymmetry, a symmetry that pairs up bosons and fermions. Although superficially the cousin is rather different from
GRAVITATIONAL POTENTIAL
y = 0
Figure 1. Particle in a potential energy well of a d + 1– dimensional gravitational theory. If the potential energy has a deep minimum as a function of the “extra” dimension with coordinate y, then a low-energy quantum particle behaves as if it were moving in the remaining d dimensions. The gauge/gravity duality makes a more far-reaching statement: Even motion along the extra dimension can be described by a d-dimensional particle theory.
28 January 2009 Physics Today
© 2009 American Institute of Physics, S-0031-9228-0901-010-2
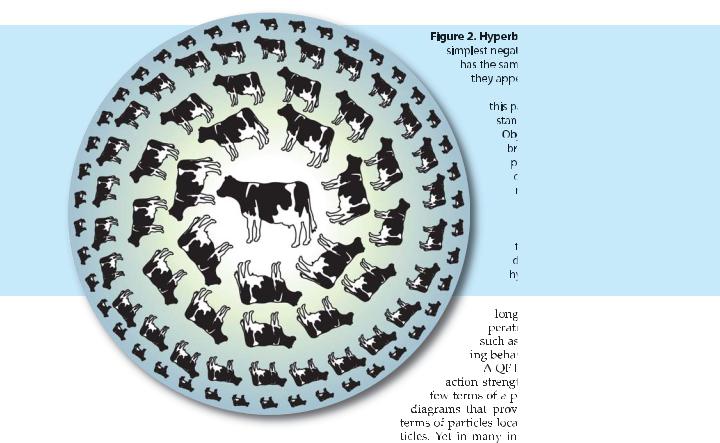
QCD, it has been useful for studying new phenomena in the physics of quarks and gluons at high temperatures, such as those that were observed not long ago at RHIC, the Relativistic Heavy Ion Collider, at Brookhaven National Laboratory.2 Similar theories have provided model-building tools for physics beyond the standard model and have served as toy models for systems with quantum critical points that appear in condensed-matter physics.
Thus theories that are solvable via the duality provide approximations to physical systems that are useful for developing intuition about strongly coupled theories. The AdS/CFT correspondence has also been valuable for elucidating some theoretical paradoxes involving the quantum behavior of black holes. In fact, it was originally discovered in investigations of such questions. The duality also gives theorists new ways to study the quantization of gravity, which is one of the important issues at the frontier of theoretical physics.
QFT is ubiquitous and difficult
Quantum field theory, which considers quantized field fluctuations, was the result of merging special relativity with quantum mechanics. It has many physical applications. In particle physics, it underlies the famous standard model, which provides a precise description of the electromagnetic, weak, and strong interactions. In statistical mechanics, QFT successfully describes second-order phase transitions that occur, for example, in the vicinity of the critical point in a water–vapor phase diagram. In that application, the quantum field fluctuations are identified with the long-range statistical fluctuations in the 3D system under study; thus the field theory is defined in three spatial dimensions without time. Generally, many-body systems are well described by QFT when the typical length scales of fluctuations are much larger than the size of the system’s atomic constituents. Instead of varying temperature or pressure, one can achieve
space. Shown here is a map of the
curved space. Each of the images
coordinate-invariant “proper” size;
to get smaller near the boundary be-
their projection onto the flat surface of page. A related phenomenon happens in standard Mercator-projection maps of Earth:
Objects of a fixed proper size appear broader at high latitudes. The map of hyperbolic space is reminiscent of M. C. Es-
cher’s Circle Limit woodcuts, but we have replaced Escher’s interlocking fish with
cows to remind readers of the physics
joke about the spherical cow as an idealization
of a real one. In the anti–de Sitter/conformal field theory correspondence, theorists have really found a hyperbolic cow.
-wavelength fluctuations at zero temby varying properties of materials the doping concentration. The result-
is called quantum criticality.
is often hard to analyze. If the interis weak, one can calculate the first perturbative expansion using Feynman a visualization of interactions in splitting or joining with other parinteresting physical problems, the cou-
pling is so strong that such an expansion is not feasible.
To further complicate matters, the coupling typically depends on the characteristic energy of a process. In QCD, perturbative computations are possible at high energies—much higher than the proton rest energy of about 1 GeV—because the coupling weakens. They become useless, however, at energies comparable to the proton rest energy. Calculations in that strongly coupled regime are difficult. One solid path toward calculating masses of hadrons such as protons or heavy mesons is to approximate continuous spacetime by a discrete lattice of points. But that approach, called lattice QCD, requires a great deal of computing power (see the article by Carleton DeTar and Steven Gottlieb in PHYSICS TODAY, February 2004, page 45). Moreover, important quantities such as transport coefficients in high-temperature QCD cannot be accessed using standard numerical approaches. Many condensed-matter problems, too, involve strongly coupled QFT. Analytic techniques that deal with strongly coupled problems are few and valuable, even if they apply to only a narrow class of theories. The gauge/gravity duality offers such a technique.
The AdS/CFT correspondence
The QFTs relevant to many applications are approximately invariant under a scaling of all d spacetime coordinates: xμ O λxμ. (The subscript μ, which runs from 0 to d − 1, indexes the particular coordinate.) For example, QCD becomes nearly scale invariant at energies high enough that the coupling constant depends only logarithmically on energy. Condensedmatter systems close to second-order phase transitions or quantum critical points are also nearly scale invariant. Theories that are invariant under scaling typically also have other symmetries, including the spacetime inversion xμ O xμ/x2 that maps the origin to infinity. Combined with the Lorentz transformations and translations of special relativity, those transformations form the so-called conformal group. A
www.physicstoday.org |
January 2009 Physics Today 29 |
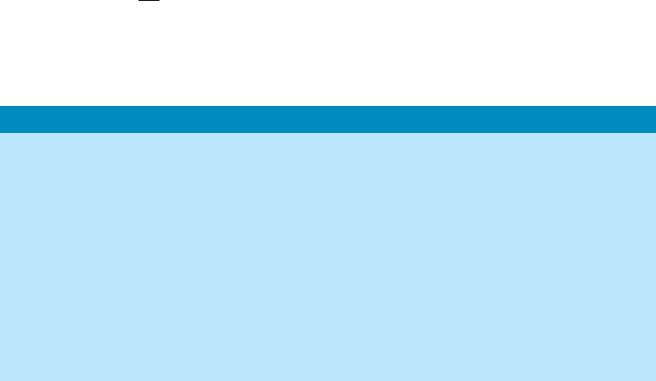
QFT invariant under that group is called a conformal field theory.
The conformal invariance is of great help in formulating an exact gauge/gravity duality because it translates into a geometrical symmetry of the dual space. If, in the QFT, all d dimensions are spatial, the dual space is the familiar d + 1–dimensional hyperbolic, or Lobachevsky, space with constant negative curvature. Figure 2 is a map of that space. If the CFT is formulated in d − 1 space dimensions and one time dimension, the unique d + 1–dimensional spacetime with the appropriate symmetry is anti–de Sitter space. For an introduction to that constant-negative-curvature spacetime, see box 1.
The intrinsic metric, or line element, of d + 1–dimensional AdS space is
ds2 = R2 [e 2y (−dx 02 + dx 12 + dx 22 + · · · + dxd2−1) + dy2], |
(1) |
where in the infinite extra dimension y ranges over all real numbers, and R is the curvature radius. The CFT corresponding to the AdS geometry is formulated in a flat d-dimensional spacetime parameterized by the coordinates x0 , . . ., xd−1. That d-dimensional spacetime may be viewed as the boundary, at y = ∞, of the d + 1–dimensional AdS space. The scale invariance of the QFT translates into a symmetry of the AdS metric under xμ O λxμ, y O y − ln λ.
What is the origin of the extra dimension in the gravitational theory? Imagine a localized state in the CFT with a given size and a position specified by the d − 1 spatial coordinates. By performing scale transformations, one can obtain states with all possible sizes, each with energy inversely proportional to the size. The extra coordinate y in the gravity theory labels the size of the state in the CFT, as shown in figure 3. More precisely, the size is proportional to e−y.
In Einstein’s gravity, the metric’s time–time component g00 (that is, the piece that multiplies dx02 in the line element) defines the gravitational potential energy V of a stationary
object of mass m: V = mc2√−g00, where c denotes the speed of light. For weak fields, g00 ≈ −(1 + 2φ/c2), with mφ the familiar Newtonian potential energy. In AdS space, V is proportional to ey, so objects are pushed to smaller y. In the dual scaleinvariant field theory, localized field configurations will thus expand to lower their energy.
Correlation functions
In a CFT, the most important physical observables are correlation functions of local operators. A two-point correlation function, for example, is a suitable average over all field configurations of a product of operators at two separated points. In a theory of phase transitions, correlation functions encode the critical exponents and other useful information.
A universal operator, present in any field theory, is the symmetric stress–energy tensor Tμν. As in electrodynamics, its time–time component T00 gives the energy density of a field, the time–space components T0i give the momentum density, and the space–space components Tij encode the pressure and shear forces.
Remarkably, to calculate correlation functions of Tμν in the dual description, one needs to consider propagation of gravitons in AdS space. The graviton carries the gravitational force, and so the d + 1–dimensional dual theory must be gravitational. Inserting Tμν(x) in a correlation function corresponds to a graviton emitted from point x on the boundary of the AdS space. Gravitons corresponding to insertions of stress–energy tensors meet in the interior and interact according to the rules of general relativity. Thus computation of the correlations of stress–energy components at different points in a field theory is translated into a problem of scattering gravitons in the interior of a curved spacetime. Other operators in the QFT correspond to other fields in the gravitational theory, and there is a one-to-one map between the two—a kind of dictionary relating the field-theory and gravity languages. The most precise correspondences found to date map CFT to string theory formulated in AdS space. String theories are described by general relativity only at low energies; at high energies, they contain a large additional set of string excitations.
In a CFT the conformal symmetry fixes the functional form of the two-point correlation function of the stress– energy tensor. But the function’s normalization, Neff, is an effective measure of the number of degrees of freedom in the CFT. In the gravity theory, the normalization is proportional to Rd−1/ħGN, where GN is Newton’s constant in d + 1 dimensions and ħ is Planck’s constant. The inverse of the normalization controls the effective gravitational coupling in the dual gravitational theory; if that theory is to be weakly coupled, the QFT must contain many degrees of freedom.
Such QFTs arise naturally in generalizations of QCD
Box 1. Anti–de Sitter space
Anti–de Sitter space is a spacetime with one time coordinate and, in general, d space coordinates. As a warm-up to looking at AdS space, however, we first present some curved twodimensional surfaces.
The 2D unit sphere, which is positively curved, is defined as the set of points in 3D Euclidean space that are equidistant from the origin: X 2 + Y 2 + Z2 = R2, where R is the radius of the sphere. (For simplicity, in the remainder of this box we consider a unitradius sphere and its analogues.) The metric, or line element, expresses an infinitesimal distance in terms of infinitesimal coordinate changes. It is simply obtained for the sphere from the metric of Euclidean space ds2 = (dX)2 + (dY)2 + (dZ)2 by restricting the coordinates to the sphere. Expressing the coordinates in terms of the standard polar and azimuthal angles, θ and φ—that is, setting X = sin θ cos φ, Y = sin θ sin φ, and Z = cos θ, one obtains the intrinsic metric of the sphere, ds2 = (dθ)2 + sin2 θ (dφ)2.
To describe a negatively curved surface, or 2D hyperbolic
space, one needs to change only some signs: X 2 + Y2 − Z 2 = −1, and ds2 = (dX)2 + (dY)2 − (dZ)2. One can define coordinates ρ and φ, through X = sinh ρ cos φ, Y = sinh ρ sin φ, and Z = cosh ρ, and obtain the intrinsic metric ds2 = (dρ)2 + sinh2 ρ (dφ)2. Note that despite the negative signs in the above definition, the metric of the 2D hyperbolic space has only positive signs. The hyperbolic space is a 2D space, not a spacetime.
Analogously, one can define a 2D AdS space by −X 2 − Y 2 + Z 2 = −1, and ds2 = −(dX)2 − (dY)2 + (dZ)2. Introducing the coordinates x0 and y defined through X = ey x0, 2Y = e−y + e y(1 − x02), and
2Z = e−y − e y(1 + x02), one finds the metric ds2 = −e2y(dx0)2 + dy2. The negative sign of the first term signifies that x0 is a time vari-
able. Equation 1 in the main text displays the metric for a d + 1– dimensional AdS space with constant curvature radius R.
In the gravity theories associated with the AdS/CFT correspondence, the important object is the AdS space itself; the flat space in which it is embedded plays no role.
30 January 2009 Physics Today |
www.physicstoday.org |

|
at |
infinity |
Boundary |
|
|
|
|
Particle in the interior
y |
Object on |
|
|
|
the boundary |
Figure 3. The extra dimension in the dual gravitational description of a conformal field theory. An object in a CFT may be pictured as being confined to the boundary of a higher-dimensional gravity theory. The blue and red disks depict two objects in the CFT that differ by only a scale transformation—that is, a uniform multiplication of all the coordinates. In the gravity theory, the two objects are described by the same particle at two different positions in the extra dimension with coordinate y. The red dot, corresponding to the bigger object on the boundary, is located at smaller y.
from three colors to a larger number of colors, N. The gluons that mediate color forces in those theories are analogues of photons, which mediate electromagnetic interactions. In contrast to uncharged photons, however, the gluons are themselves colored, and their number is N2 − 1. Thus Neff is comparable to N2.
But large Neff alone is not enough to guarantee a simple gravity description. Gravity theories do not include light particles with large spin. On the other hand, in a weakly coupled QFT, one often has operators with large spin. The mismatch may be removed by turning up the interaction strength g, the QFT analogue of the electric charge e in electrodynamics. To see that a stronger interaction simplifies the dual gravitational theory, note that the curvature radius of AdS space is proportional to (g2N)1/4 multiplied by the fundamental stringtheory length scale. Thus the spacetime in a gravitational theory that is dual to a field theory with g2N 1 is weakly curved and one can reliably study the gravitational theory with the methods of general relativity. Conversely, if the field theory coupling is weak—that is, g2N 1—then the radius of curvature is smaller than the string length. In that regime, general relativity is not a good approximation.
The most tractable correspondences therefore involve strongly coupled CFTs with many color states. Luckily, theorists have constructed many such theories. The simplest and most studied of them is the gauge theory in four spacetime dimensions with the greatest possible number of supersymmetries that pair up bosonic and fermionic fields. Although supersymmetry is not fundamental to gauge/gravity duality, it is a technical tool that simplifies its analysis.
Black holes and thermal field theories
A fascinating aspect of general relativity is the existence of black holes, which are surrounded by event horizons. Any classical object that falls through such a horizon cannot return, but Stephen Hawking demonstrated that the horizon itself radiates with a characteristic temperature T. Now sup-
www.physicstoday.org
pose that far away from the black hole—in higher dimensions it would be a black membrane—the d + 1–dimensional spacetime looks like AdS space. One can then consider a black membrane with a horizon located at a fixed position y0 and extended in the d − 1 spatial dimensions, as illustrated in figure 4. The gravitational theory in the black membrane spacetime is again described by a d-dimensional QFT, but now the QFT has been heated up—to the same temperature T as the horizon!
The duality connecting black holes with strongly interacting QFTs has many interesting implications. As shown by Jacob Bekenstein and Hawking, a black hole has an entropy proportional to the area of its event horizon. Computing the finite-temperature entropy for a strongly interacting QFT is generally difficult, since particle interactions make important contributions to the free energy and therefore to the entropy. Yet when the QFT has a dual gravity theory, its entropy can be found simply through calculating the area of the horizon.
What does the “blackness” of the horizon correspond to in the QFT? Black holes are excellent particle absorbers. Any fluctuation of the black hole geometry decays exponentially, since waves near the horizon are swallowed by the black hole. In the finite-temperature QFT, that phenomenon corresponds to the rapid thermalization of fluctuations.
Thermodynamic and transport properties of a strongly coupled QFT are related to properties of the black hole geometry. Thus the computation of a transport coefficient, such as the shear viscosity, becomes tractable in the gravitational for- mulation—one has to solve a certain wave equation in the black hole geometry. Calculations along those lines have been used to gain intuition about complicated finite-temperature QCD problems that can be realized experimentally. RHIC is believed to have produced a new state of matter, which was
Horizon |
|
at |
infinity |
Boundary |
|
||
|
|
|
Ripple on |
Long-range |
the horizon |
density |
|
fluctuation |
y0 |
y |
Figure 4. The black hole duality. A strongly interacting quantum field theory at a finite temperature is related to a black membrane—the higher-dimensional analogue of a black hole—in the dual gravity system. Thermodynamic properties of the strongly coupled QFT are related to geometric properties of the horizon. For example, a long-range fluctuation in the density of the thermal system corresponds to a ripple on the event horizon surrounding the black membrane. This ripple is absorbed by the black membrane; the corresponding phenomenon in the thermal QFT is thermalization.
January 2009 Physics Today 31
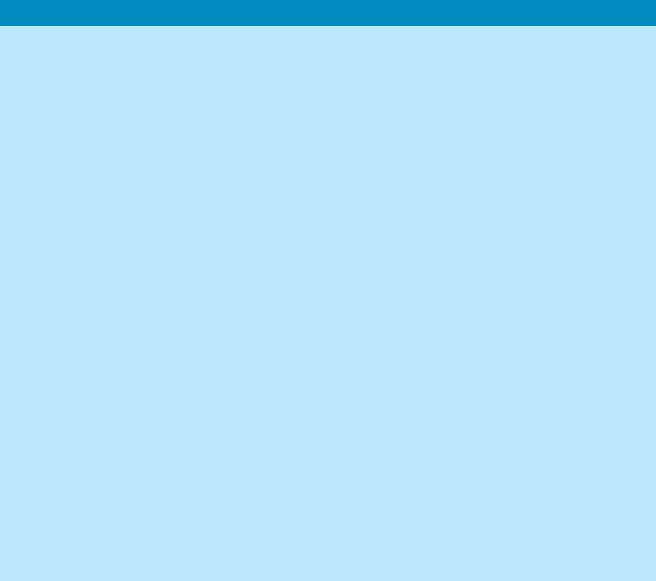
originally dubbed the quark–gluon plasma. Experiments at RHIC established that the matter behaves more like a liquid than a plasma because its viscosity is much smaller than had been expected.2 Interestingly, when the gauge/gravity duality is applicable, it also predicts a low value for the viscosity (see reference 3 and PHYSICS TODAY, May 2005, page 23). One can compute other quantities, such as the energy loss that a very energetic quark will suffer as it propagates through the medium. In some cases the finite-temperature gauge/gravity duality has motivated the study of new strong-coupling phenomena that may be experimentally observable.
A confining potential-energy well
Gauge/string duality also gives new insights into color confinement, a deep and mysterious aspect of QCD. Even though QCD is formulated in terms of the fundamental colored par- ticles—quarks and gluons—nobody has observed a free one; they are always confined inside color-neutral particles such as protons, neutrons, and pions. The way in which hadrons are made of quarks and gluons is quite different from the way in which nuclei are made of neutrons and protons. Those nuclear constituents are often bound together tightly, but hitting the nucleus hard enough will liberate them. No matter
how hard one hits a hadron, though, it will decay only into other hadrons, not into free quarks and gluons. That phenomenon has numerical support from lattice simulations of gauge theory, but its theoretical proof is still missing.
Once again, gauge/string duality provides new insights. Admittedly, no simple string theory dual to QCD has been found, but some confining gauge theories do have tractable dual formulations.4 Those theories are not scale invariant; they have a preferred length scale that is comparable to the size of the lightest bound states. For example, in QCD the scale is about that of the proton radius, 10−15 m. When the QFT is not scale invariant, the dual spacetime has nonconstant curvature and a “warped” metric of the form
ds2 = e 2A(y) (−dx 02 + dx 12 + · · · + dxd2−1) + dy2. |
(2) |
In such a spacetime, the gravitational energy of a massive particle is proportional to eA(y). The function A(y) is such that for large y, the energy approaches the exponential ey found in the AdS metric, but its form becomes more complicated as y decreases. It attains its absolute minimum, eA(0), at y = 0. Thus massive objects fall to the bottom of the gravita-
Box 2. String theory and QCD
Relations between string theory and strong interactions are older than quantum chromodynamics (QCD) itself. In fact, string theory was born in the late 1960s to describe hadronic spectra and interactions. The motivation was partly experimental. Some observed hadronic states exhibited a linear relation between mass squared and spin; relativistic strings could explain that relation. But it did not take long for the string models to run into problems: The simplest string theories could be defined only in 10 spacetime dimensions (for theories containing bosons and fermions) or 26 dimensions (for theories with bosons only), and they had to include quantum gravity.8
Furthermore, deep inelastic scattering experiments were showing signs of more fundamental constituents inside the hadrons, a result that seemed incompatible with a string description. Those experiments provided an important piece of information: Strong interactions become weaker at high energies. The only known theories in four spacetime dimensions with that property, called asymptotic freedom, are the Yang–Mills gauge theories (see PHYSICS TODAY, December 2004, page 21). In the early 1970s, the SU(3) Yang–Mills gauge theory (that is, QCD) was proposed as the exact description of strong interactions, and it is now an integral part of the standard model. Yet QCD is hard to solve because the coupling g becomes strong at low energies.
Strings, though, may come to the rescue. In QCD they form as color electric flux tubes that are well established through numerical lattice simulations. Also, QCD itself simplifies if the number of colors is changed from three to a large number N, provided that g2N is held fixed.9 A simple argument shows that in the large-N limit, the gauge theory should become a kind of string theory, with splitting and joining interactions suppressed by 1/N. The argument, however, does not specify the string theory. Theorists initially assumed that the string theory should be defined in the four spacetime dimensions of the gauge theory. But Alexander Polyakov later conjectured that one needs at least five dimensions and a curved spacetime.10
Meanwhile, theorists continued to study string theory as a theory of quantum gravity. They intently studied 10D string theory and came to understand many of its properties in great
detail. In the mid-1990s Joseph Polchinski found that the theory contains various spatially extended objects called D-branes.11 One type of D-brane has three infinite spatial dimensions, just like the observed world. At low energies, N coincident D-branes of that type are described in terms of a 4D SU(N) gauge field theory similar to QCD, except with extra sym- metries—supersymmetry and conformal invariance. But the stack of D-branes curves the 10D spacetime in which it is embedded. In the region near the stack, half of the dimensions form a 5D anti–de Sitter space, and the rest describe a 5D sphere. So, one point of view on the dynamics of the D-branes uses the 4D gauge theory; the other, the 10D string theory in the curved space (see PHYSICS TODAY, August 1998, page 20). The conjecture that the two theories are exactly equivalent provides the most solid example of the gauge/string duality. Recent tests of the correspondence12 involve calculations of certain quantities in the supersymmetric gauge theory as functions of g2N. Remarkably, they rely on Bethe ansatz methods that are frequently used to study spin chains arising in con- densed-matter physics (see the article by Murray Batchelor, PHYSICS TODAY, January 2007, page 36). For large g2N, the results agree with the predictions of string theory.
Similar gauge/string dualities have been found for theories that are not scale invariant, but QCD itself has so far evaded a solution. Due to asymptotic freedom, QCD is weakly coupled at high energy. The dual description of weakly coupled theories requires highly curved spacetimes for which higher powers of curvature are more important than the leading power. At high energy, though, one doesn’t need to resort to the dual calculation; the theory is weakly coupled. On the other hand, at low energies the coupling is strong enough to invalidate naive perturbative computations, but not so strong that QCD has a weakly curved dual description. General relativity is thus not enough to analyze the dual theory; one needs the full apparatus of string theory, which has so far been too difficult to implement. Nonetheless, the tools developed during the past 10 years or so have generally improved physicists’ understanding of strongly coupled gauge theory.
32 January 2009 Physics Today |
www.physicstoday.org |
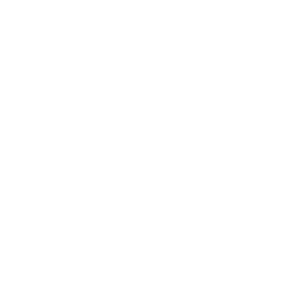
tional well, where they retain a nonzero energy proportional to eA(0). Note that in QCD most of the proton mass comes from the effects of confinement; the masses of the quarks make a relatively small contribution.
Theorists have posited that as a heavy quark–antiquark pair separates in a confining theory, a color electric flux tube forms with constant energy per unit length and a characteristic thickness. That object, often called a confining string, has been observed in numerical simulations. When such a string is spinning, it nicely explains the masses of high-spin mesons. (For more on how string theory is connected to QCD, see box 2.) How is the confining flux tube manifested in the dual, string-theoretic description of the gauge theory? The answer is unexpectedly simple: It is the fundamental string located at the bottom of the potential-energy well shown in figure 5. Indeed, the energy per unit length of a string at fixed y and stretched along one of the spatial directions in the metric of equation 2 is proportional to Ts e2A(y), where Ts is the funda- mental-string tension. Such a string naturally falls to the bottom of the gravitational potential-energy well, where it retains a nonvanishing tension Ts e2A(0). Since all confining gauge theories are expected to generate flux tubes, their dual formulations cannot be given merely in terms of a gravitational field theory—they must involve string theory.
A variety of applications
The gauge/gravity duality has found theoretical applications to physics ranging from the highest to the lowest conceivable energies. At the high end, theorists are exploring physics at the Planck scale of about 1019 GeV, which necessarily involves quantized fluctuations of spacetime geometry. Gauge theories with N colors are dual to quantum gravity in curved spacetime, with the effective gravitational coupling being of order 1/N. Those gauge theories have conventional quantum mechanics, with unitary time evolution of wavefunctions. Therefore, the duality provides the most solid argument to date for why quantum gravity in general, and a black hole in particular, does not destroy information.
At the low-energy end, QFTs often arise in the lowtemperature domain of condensed-matter physics. One much-explored class of examples concerns the behavior of systems at quantum critical points.5 The relevant zerotemperature, scale-invariant theories are analogous to field theories describing second-order phase transitions, but they are formulated in d spacetime dimensions rather than in just spatial dimensions. In quantum critical systems, one is interested in computing transport properties at zero or finite temperatures. The theories with tractable dual gravity descriptions can be viewed as toy models for which such computations are feasible at strong coupling. So far, those manageable theories don’t correspond to real-world con- densed-matter systems, but they may capture some of those systems’ important features. For a study of transport properties near quantum criticality in that vein, see reference 6.
Now is an exciting time in particle physics—the Large Hadron Collider at CERN will soon reach its peak collision energy of 14 TeV. We are confident that the current standard model of particle physics is not the final word about nature at high energies, and the LHC’s experiments may well shed light on the new layer of physics. Those who build particle models explore many different scenarios, but they are often limited by difficulties associated with strongly coupled QFT. The gauge/gravity duality enables them to investigate alternative, strongly coupled theories. And in fact, the warped AdS-like geometries of equation 2 were also introduced for a number of phenomenological reasons, in particular to ex-
Gravitational
potential
String
|
at |
infinity |
Boundary |
|
|
|
|
y = 0 |
y |
Figure 5. A string theory of quark confinement. A long fundamental string that has fallen to the bottom of the gravitational potential energy well describes the so-called color flux tube in a confining gauge theory at the boundary of the extra dimension. Such strings explain why the potential energy between a quark and an antiquark rises linearly with their separation and prevents the two particles from escaping to freedom. The thickness of the string in the gauge theory is related to the position of the fundamental string in the y direction.
plain why the scale of weak interactions, 250 GeV, is so much smaller than the Planck scale.7
The gauge/gravity duality has enabled field theorists to explore new possibilities away from weak coupling. Some strongly coupled field theories can now be solved via their dual curved spacetimes and provide a “hyperbolic cow” approximation to interesting physical systems. We are optimistic that the future will reveal even closer connections between gauge/gravity duality and nature.
References
1.J. M. Maldacena, Adv. Theor. Math. Phys. 2, 231 (1998); J. Maldacena, Int. J. Theor. Phys. 38, 1113 (1999); S. S. Gubser, I. R. Klebanov, A. M. Polyakov, Phys. Lett. B 428, 105 (1998); E. Witten, Adv. Theor. Math. Phys. 2, 253 (1998).
2.I. Arsene et al., BRAHMS collaboration, Nucl. Phys. A 757, 1 (2005); B. B. Back et al., PHOBOS collaboration, Nucl. Phys. A 757, 28 (2005); J. Adams et al., STAR collaboration, Nucl. Phys. A 757,
102 (2005); K. Adcox et al., PHENIX collaboration, Nucl. Phys. A 757, 184 (2005).
3.G. Policastro, D. T. Son, A. O. Starinets, Phys. Rev. Lett. 87, 081601 (2001).
4.I. R. Klebanov, M. J. Strassler, J. High Energy Phys. 2000(08), 052 (2000).
5.S. Sachdev, Quantum Phase Transitions, Cambridge U. Press, New York (1999).
6.C. P. Herzog, P. Kovtun, S. Sachdev, D. T. Son, Phys. Rev. D 75, 085020 (2007).
7.L. Randall, R. Sundrum, Phys. Rev. Lett. 83, 3370 (1999).
8.T. Yoneya, Lett. Nuovo Cimento. 8, 951 (1973); J. Scherk, J. H. Schwarz, Nucl. Phys. B 81, 118 (1974).
9.G. ’t Hooft, Nucl. Phys. B 72, 461 (1974).
10.A. M. Polyakov, Nucl. Phys. B Proc. Suppl. 68, 1 (1998).
11.J. Polchinski, Phys. Rev. Lett. 75, 4724 (1995).
12.N. Beisert, B. Eden, M. Staudacher, J. Stat. Mech. 2007, P01021
(2007). |
|
www.physicstoday.org |
January 2009 Physics Today 33 |
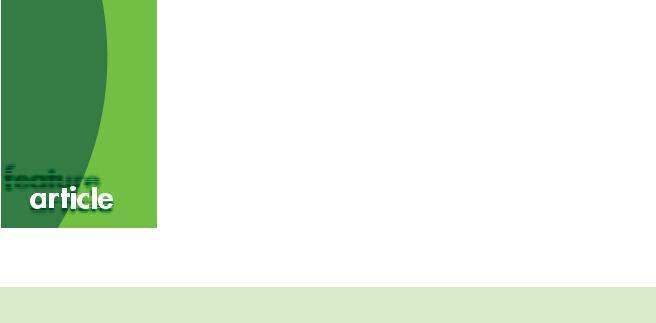
Carbon nanotube electronics and
feature photonics
Phaedon Avouris
Their geometry, mechanical flexibility, and unique charge-transport properties make carbon nanotubes ideally suited to supplant silicon in the next generation of FETs.
Phaedon Avouris is an IBM fellow and manager of nanometer-scale science and technology at the IBM Thomas J. Watson Research Center in Yorktown Heights, New York.
Since the demonstration of the first transistors in 1947, computing electronics has been based mostly on one material, silicon. The emergence of MOSFETs in the 1960s and the development of microlithography and other fabrication techniques have allowed the continuous miniaturization of silicon devices. The resulting increases in density and switching speed and the reduced cost have made today’s information age possible. Unfortunately, for various technological and even fundamental physical reasons, that scaling process is reaching its useful limits. In response, researchers are exploring ideas for logic devices based on either different operating principles or different materials whose properties can sustain the scaling to yet smaller size and greater performance.
Among the alternative technologies, carbon-based electronics is particularly significant. It is based on the 1991 discovery of carbon nanotubes1 and the more recent studies of individual graphite layers, so-called graphenes.2 A singlewalled CNT can be thought of as a ribbon of graphene rolled into a seamless, one-atom-thick cylinder. The ribbon’s width determines the CNT’s diameter (typically 1–2 nm), and the direction in which the graphene is rolled defines the CNT chirality. Moreover, most of the CNT’s electrical properties can be deduced from those of graphene after additional boundary conditions are introduced.3 Both materials display outstanding electrical properties such as either ballistic transport or diffusive transport with long mean free paths. And both are mechanically strong, flexible, and thermally conductive. But unlike graphene, semiconducting nanotubes have fairly large bandgaps that allow them to produce large on/off current ratios when fashioned into logic devices such as an FET.
The structure of traditional MOSFETs is described in the box on page 35. In a CNT FET, the silicon channel is replaced by an individual single-walled CNT (see figure 1); the first such transistors were independently reported in 1998 from groups at Delft University of Technology and IBM’s Thomas J. Watson Research Center (see the article by Cees Dekker in PHYSICS TODAY, May 1999, page 22).4
For the last several decades optoelectronics has been dominated by III–V semiconductors, particularly gallium arsenide and its alloys. In 2002, Rice University researchers observed photoluminescence from CNTs,5 and the following year, IBM researchers reported electroluminescence.6 Those later developments opened the possibility of basing both computing elec-
tronics and optoelectronics on the same material; for recent reviews, see reference 3.
Nanotube transistors
A CNT has an atomic and electronic structure that gives it unique advantages as an FET channel. Its small, 1- to 2-nm diameter enhances the gate’s ability to control the potential of the channel, particularly when the gate is configured to wrap around the CNT. The strong coupling makes the CNT the ultimate thin-body semiconductor system and allows one to shrink an FET in size yet still avoid the dreaded “shortchannel effects,” in which the gate field basically loses control of the device.
That all bonds in the CNT are satisfied and the surface is atomically smooth also has important implications. Absent are the scattering of electrons by surface states and the roughness that plagues conventional FETs, especially at high gate voltages. The electrical bandgap of a semiconducting CNT is inversely proportional to the tube diameter,3 which allows such tubes to be used in various different applications. As discussed later, the key advantage is the CNT’s high charge mobility μ and its low capacitance. For typical performance characteristics as an FET channel, see figure 2.
The “simple” substitution of a silicon channel with a CNT dramatically alters the detailed physics of the device. The CNT’s quasi-one-dimensional character, strong electron confinement, nanometer width, and strong covalent bonding drastically affect the channel’s electricaland thermaltransport properties. The chemistry of the metal–carbon contacts, the interaction of insulators with CNTs, and the sensitivity of those one-atom-thick structures to environmental influences all raise fascinating challenges and opportunities.
Carrier scattering
A key reason to use CNTs in electronics is their excellent transport properties. The elastic scattering of charge carriers is weak, and the carrier mean free paths are long—on the order of a micrometer for pure CNTs.3 Inelastic phononscattering processes therefore tend to dominate. The strength of the scattering depends on the energy of the carriers. At low temperatures and low bias, only acoustic phonons can couple to electrons, which gives rise to an inverse temperature dependence of the carrier mobility in semiconducting CNTs. The weak temperature dependence in quasi-one dimension is due to phase-space restrictions
34 January 2009 Physics Today
© 2009 American Institute of Physics, S-0031-9228-0901-020-5
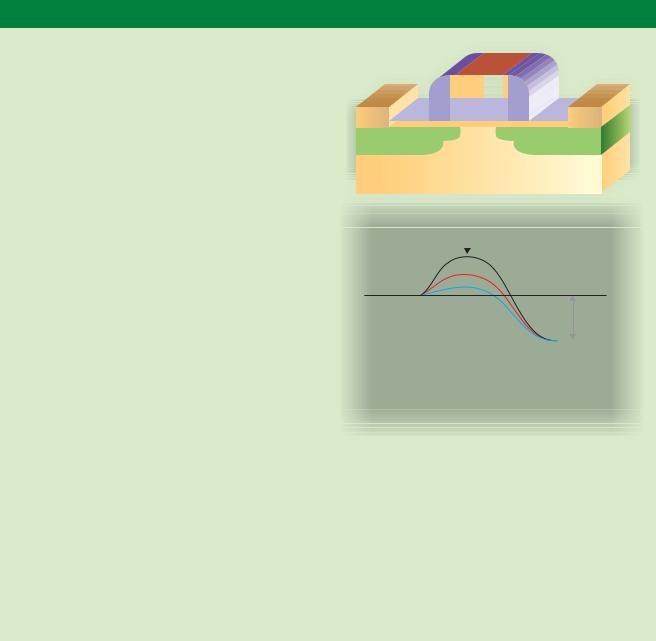
The principle of a MOSFET
The basic structure of a MOSFET involves a channel made of a semiconductor, typically silicon, connected to two electrodes: a source and drain. An insulating thin film, usually silicon dioxide, separates the channel, source, and drain from a third electrode called the gate (G), as pictured schematically. By applying a voltage Vgs between the gate and source, the conductance of the semiconducting channel can be modulated. Charge carriers (electrons or holes) traveling between source and drain encounter a materialand structure-dependent energy barrier in the bulk of the semiconductor.
The barrier, shown in the energy profile as a function of the channel position, lies in the conduction band for an electron (the charge carrier in an n-type semiconductor) or in the valence band for a hole (the carrier in a p-type semiconductor). The electric field generated by the biased gate, depending on its direction and strength, lowers or raises the barrier and thus changes the conductivity. For example, a positive gate voltage lowers the barrier for an electron and a negative gate voltage raises it. The drain–source voltage Vds provides the driving force that moves carriers across the channel.
The scaling of a MOSFET involves reducing its dimensions and supply voltage by the same factor α. The speed of the device thus increases by α and the density of devices, when packed together, by α2, while the power density remains constant.
A number of limitations arise as dimensions reach the nanometer regime. Two variables are already close to their scaling limits. One of them is the thickness of the gate insulator, typically SiO2, which is currently about 1 nm. Were that thickness to shrink much more, the leakage current from quantum tunneling would undermine the switching action of the FET. The leakage current also contributes to a major problem—excess power dissipation.
Another parameter that is difficult to scale down is the operating voltage V usually about 1 V. The dynamic switching energy
of a device is given by ½(Cdev + Cw)V 2, where Cdev and Cw are the device and wiring capacitance contributions, respectively. If V is
not reduced as devices shrink in size and are more closely packed, the dissipated power density will increase. High temperatures then develop locally and decrease both the performance and lifetime of devices. Ways to reduce V and the total capacitance are, therefore, being vigorously pursued.
To maintain the basic principle of the FET—that is, the con-
|
|
|
|
|
|
|
|
|
|
|
|
|
|
|
|
|
|
|
|
|
|
Gate |
|
|
|
|
|
|
|
|
|
|
|
|
|
|
|
|
|
|
|
|
|
|
|
|
|
|
|
Gate insulator |
|
|
|
|
|
||||
|
|
Source |
|
Channel |
Drain |
|||||||||
|
|
|
|
|
|
|
|
|
|
|
||||
|
|
Substrate |
|
|
|
|
|
|
|
|
|
|
||
|
|
|
|
|
|
|
|
|
|
|
|
|
|
|
|
|
|
ENERGY |
|
|
|
Increasing Vgs |
|
|
|
|
|
||
|
|
|
|
|
|
|
|
|
|
|
||||
|
|
|
|
|
|
|
|
|
|
|
||||
|
|
|
|
|
|
|
|
|
|
|
|
|
|
|
|
|
|
|
|
|
|
|
|
|
|
|
|
|
|
|
|
Fermi |
|
|
|
|
|
|
|
|
|
|
|
|
|
|
energy |
|
|
|
|
|
|
|
|
e·Vds |
|
||
|
|
|
|
|
|
|
|
|
|
|
|
|
||
|
|
|
|
|
|
|
|
|
|
|
|
|
|
|
|
|
|
|
|
|
|
|
|
|
|
Fermi |
|
|
|
|
|
|
|
|
|
|
|
|
|
|
energy |
|
|
|
|
|
Source |
|
|
Channel |
|
Drain |
|
|
|||||
|
|
|
|
|
|
|
|
|
|
|
|
|||
|
|
|
|
|
|
POSITION |
|
|
|
|
|
|||
|
|
|
|
|
|
|
|
|
|
|
|
|
|
|
|
|
|
|
|
|
|
|
|
|
|
|
|
|
|
trol of transport in the channel using gate bias—one also needs to maintain a certain relationship between the screening length λ—the scale over which the electric file is screened out in the semiconductor—and the length of the gate, LG. Specifically, LG should be a few times λ, which depends on the channel and oxide-insulator thicknesses dC and dox, respectively, and their dielectric constants, εC and εox. In a planar FET, λ = (dCdoxεC/εox)1/2. Therefore, thin-dC devices with thin, high-εox dielectrics are desired. To increase the effective coupling of the gate and channel, a geometry in which the metal gate wraps around the channel is optimal. In that cylindrical geometry, λ is shorter and depends less on dox. Consequently, devices can be made shorter, and the scaling process can continue.
and the absence of low-angle scattering.
As a result of energyand momentum-conservation requirements and the mismatch between the phonon sound velocity and electron band velocity, only a small fraction of phonons can effectively participate in the scattering in CNTs. The consequence is a very high low-electric-field mobility (typically about 10 000 cm2/Vs), and values as high as 100 000 cm2/Vs have been reported, even at room temperature, by the University of Maryland’s Michael Fuhrer. That is unlike other materials such as III–V semiconductors, whose charge mobilities are high at low temperatures but substantially degraded at room temperature.
Unlike acoustic-phonon scattering, optical-phonon scattering is very strong in CNTs. The phonons contract and elongate the carbon–carbon bonds, thus strongly modulating the electronic structure and coupling the electrons and phonons. At ambient temperatures, however, the phonon population is negligible, and electrons must have energies larger than
www.physicstoday.org
about 160 meV to emit such a phonon. That can only be achieved under high voltage-bias conditions.
Unusual, nonequilibrium phonon distributions can be produced by current flow in nanotubes at those high biases due to differences in electron–phonon coupling among the various phonon modes, the large disparity in phonon frequencies between acoustic and optical modes, and the poor thermal interface between the CNT and the insulating substrate. As the carrier energy increases further, other inelastic processes take place that involve CNT excited states, which are discussed later.
Current injection
The semiconducting CNTs forming FET channels are connected to metal electrodes, typically palladium, gold, titanium, or aluminum. The differences in the metal and CNT work functions lead to charge transfer at their interface. The resulting interface dipoles produce a potential-energy
January 2009 Physics Today 35
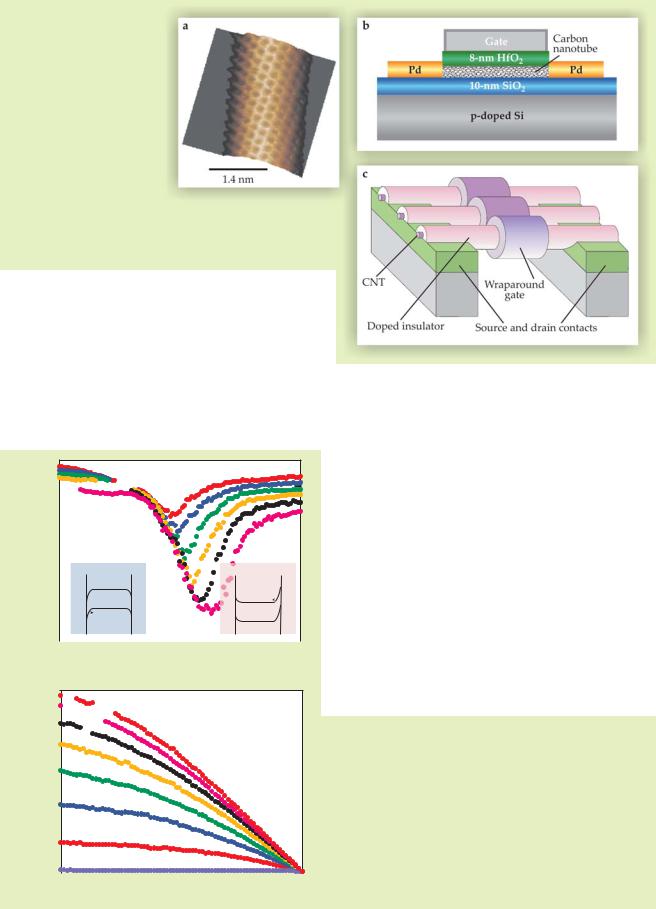
Figure 1. A single-walled carbon nanotube, shown in this scanning tunneling microscope image (a), can form the basis of an FET. (b) In a top-gated configuration, a single nanotube is sandwiched between two insulating layers, hafnium oxide and silicon dioxide in this case. Palladium metal electrodes serve as source and drain. (Adapted from A. Javey et al., Nano Lett. 4, 1319, 2004.) (c) In an alternate config-
uration, an insulating layer and metal gate are wrapped around each nanotube to optimize the gate–channel coupling. The nanotube segments away from the gate are doped. (Adapted from Ph. Avouris et al., ref. 3.)
must equal the bandgap—the FET operates as a unipolar device, meaning it transports only one type of carrier, electrons or holes. For example, a metal such as Pd with a high work function could be used to form a nearly barrierless contact
a 10−6
10−7
(A) |
10−8 |
|
|
|
|
|
|
|
|
|
|
|
|
|
|
|
|
|
|
|
|
|
|
|
|
|
|
|
|
|
|
|
|
|
|
|
|
|
|
|
|
|
|
|
|
|
|
||
|
|
|
|
|
|
|
|
|
|
|
|
|
|
|
|
|
|
|
|
|
|
|
|
|
CURRENT |
10−9 |
|
|
|
|
|
|
|
|
|
|
|
|
|
|
|
|
|
|
|
|
|
|
|
|
|
|
|
|
|
|
|
|
|
|
|
|
|
|
|
|
|
|
|
|
|
|||
10−10 |
|
|
|
|
|
Vds < 0 V |
|
|
|
|
|
|
|
|
Vds < 0 V |
|
|
|
|
|
||||
|
|
|
|
|
|
|
Vgs < 0 V |
|
|
|
|
|
|
|
|
Vgs > 0 V |
|
|
|
|
|
|||
|
10−11 |
|
|
|
|
|
|
|
|
|
|
|
|
|
|
|
Electrons |
|
|
|
|
|
||
|
|
|
|
|
|
|
|
|
|
|
|
|
|
|
|
|
|
|
|
|||||
|
|
|
|
|
|
|
|
|
|
|
|
|
|
|
|
|
|
|
|
|
|
|
|
|
|
|
|
|
|
|
|
|
|
|
|
|
|
|
|
|
|
|
|
|
D |
||||
|
10−12 |
|
|
|
|
|
Holes |
D |
|
|
|
|
|
|
|
|
||||||||
|
|
|
|
|
|
|
|
|
|
S |
|
|
|
|||||||||||
|
|
|
|
|
|
|
|
|
|
|
|
|
|
|
|
|
|
|||||||
|
|
|
|
|
|
|
|
|
|
|
|
|
|
|
|
|
|
|||||||
|
|
|
|
S |
|
|
|
|
|
|
|
|
|
|
|
|
|
|
|
|
||||
|
10−13 |
|
|
|
|
|
|
|
|
|
|
|
|
|
|
|
|
|
|
|
|
|
|
|
|
|
|
|
|
|
|
|
|
|
|
|
|
|
|
|
|
|
|
|
|
|
|
|
|
|
|
|
|
|
|
|
|
|
|
|
|
|
|
|
|
|
|
|
|
|
|
|
|
|
|
−1.0 |
|
|
−0.5 |
0.0 |
0.5 |
1.0 |
1.5 |
|
2.0 |
GATE VOLTAGE Vgs (V)
b16
14
12
(μA) |
10 |
|
|
|
|
|
|
|
|
|
|
|
|
|
|
|
|
|
|
|
|
|
|
|
|
|
|
|
|
|
|
|
|
|
|
|
|
|
|
|
|
|
|
|
|
|
|
|
|
|
|
|
|
|
|
|
|
|
|
|
|
|
|
|
|
|
|
|
|
|
|
|
|
|
|
|
|
|
|
|
|
|
|
|
|
|
|
|
|
|
|
|
|
|
|
|
|
|
|
|
|
|
|
|
|
|
|
|
|
|
|
|
|
|
|
|
|
|
|
|
|
|
|
|
|
|
|||
CURRENT |
8 |
|
|
|
|
|
|
|
|
|
|
|
|
|
|
|
|
|
|
|
|
|
|
|
|
|
|
|
|
|
|
|
|
|
|
|
|
|
|
|
|
|
|
|
|
|
|
|
|
|
|
|
|
|
|
|
|
|
|
|
|
|
|
|
|
|
|
|
|
|
|
|
|
|
|
|
|
|
|
|
|
|
|
|
|
|
|
|
|
|
|
|
|
|
|
|
|
|
|
|
|
|
|
|
|
|
|
|
|
|
|
|
|
|
|
|
|
|
|
|
|
|
|
|
|
|
|
|
|
|
6 |
|
|
|
|
|
|
|
|
|
|
|
|
|
|
|
|
|
|
|
|
|
|
|
|
|
|
|
|
|
|
|
|
|
|
|
|
|
|
|
|
|
|
|
|
|
|
|
|
|
|
|
|
|
|
|
|
|
|
|
|
|
|
|
|
|
|
|
|
|
|
|
|
|
|
|
|
|
|
|
|
|
|
|
|
|
|
|
|
|
|
|
|
|
|
|
|
|
|
|
|
|
|
|
|
|
|
|
|
|
|
|
|
|
|
|
|
|
|
|
|
|
|
|
|
|
|
||
|
4 |
|
|
|
|
|
|
|
|
|
|
|
|
|
|
|
|
|
|
|
|
|
|
|
|
|
|
|
|
|
|
|
|
|
|
|
|
|
|
|
|
|
|
|
|
|
|
|
|
|
|
|
|
|
|
|
|
|
|
|
|
|
|
|
|
|
|
|
|
|
|
|
|
|
|
|
|
|
|
|
|
|
|
|
|
|
|
|
|
|
|
|
|
|
|
|
|
|
|
|
|
|
|
|
|
|
|
|
|
|
|
|
|
|
|
|
|
|
|
|
|
|
|
|
|
|
|
||
|
2 |
|
|
|
|
|
|
|
|
|
|
|
|
|
|
|
|
|
|
|
|
|
|
|
|
|
|
|
|
|
|
|
|
|
|
|
|
|
|
|
|
|
|
|
|
|
|
|
|
|
|
|
|
|
|
|
|
|
|
|
|
|
|
|
|
|
|
|
|
|
|
|
|
|
|
|
|
|
|
|
|
|
|
|
|
|
|
|
|
|
|
|
|
|
|
|
|
|
|
|
|
|
|
|
|
|
|
|
|
|
|
|
|
|
|
|
|
|
|
|
|
|
|
|
|
|
|
||
|
0 |
|
|
|
|
|
|
|
|
|
|
|
|
|
|
|
|
|
|
|
|
|
|
|
|
|
|
|
|
|
|
|
|
|
|
|
|
|
|
|
|
|
|
|
|
|
|
|
|
|
|
|
|
|
|
|
|
|
|
|
|
|
|
|
|
|
|
|
|
|
|
|
|
|
|
−1.2 |
|
−0.8 |
|
−0.4 |
0.0 |
||||||||||||||||||||||||||||||||||||||||||||||||
|
|
|
|
|
|
|
|
|
|
|
|
|
|
DRAIN–SOURCE VOLTAGE Vds (V)
for holes. But electron injection would then experience the maximum barrier with a value close to the bandgap. Ali Javey and colleagues at Stanford University used Pd as a metal in contact with 2-nm-diameter CNTs and observed quasiballistic transport in short, p-type CNT FETs.8
In general, the current strongly depends not only on the type of metal but also on the diameter of the CNT. Moreover, because carrier transport through the metal–CNT interface is dominated by quantum mechanical tunneling through the SB, the barrier’s thickness becomes critical. But thanks to the enhanced screening provided by a metal gate near the thin oxide layer, both SBs can be made thin enough to allow the injection of either electrons, holes, or both simultaneously.7 That type of transistor is called ambipolar. The insets of figure 2 show how the application of different gate voltages triggers the injection of electrons or holes. The SB thickness can be modified by doping the CNT near the contacts or by using CNT FETs with multiple gates.
The 1D character and single-atomic-layer structure of CNTs make them susceptible to environmental perturbations. Intrinsic screening is weak, and trapped charges in a gate insulator shift electrical characteristics such as the FET threshold voltage. Specific interactions such as charge trans-
Figure 2. Charge-transport characteristics. (a) The current Id versus gate voltage Vgs measured from an ambipolar carbon nanotube FET as the drain–source voltage Vds decreases (bottom to top) from −0.1 V to −1.1 V in steps of -0.2 V. The left inset shows a schematic band structure of a CNT FET under negative gate bias and negative drain–source bias. Holes are injected into the CNT from the source (S). The right inset shows the reverse: Under positive gate bias, electrons are injected from the drain (D). (b) Output characteristics of a CNT FET plotted as Id vs. Vds. From bottom to top, Vgs varies from +1 V, the off state of the FET, to −2.5 V, the on state, in steps of −0.5 V. (Adapted from Ph. Avouris et al., ref. 3.)
36 January 2009 Physics Today |
www.physicstoday.org |
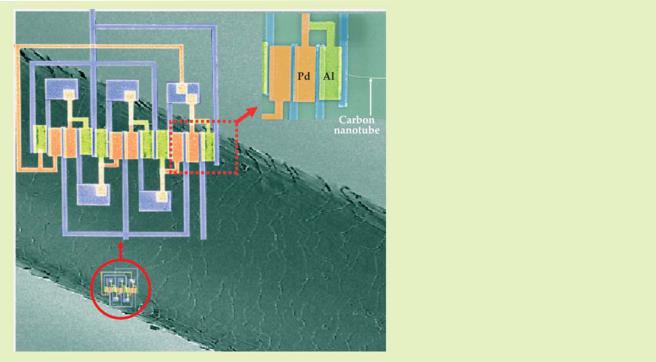
fer to or from the substrate or the absorption of species on the CNT, the metal electrodes, or the substrate can alter the character of the transport, the doping, and the resistance.3 Indeed, such effects on the electrical properties of CNTs provide the basis for various sensor applications.
Implementation and performance
Early CNT FETs were simple structures composed of a long CNT channel that is van der Waals–bonded to metal contacts and back-gated using the Si substrate itself.3,4 Although functional, they had rather poor electrical characteristics. Shalom Wind and colleagues improved on those characteristics by embedding the CNTs in the metal electrodes and using top metal gates and thin gate insulators. Javey, Hongjie Dai, and coworkers further enhanced the channel–gate coupling and performance by using high-dielectric insulators such as zirconium oxide.8 A number of different device structures have since evolved, among them multiple-gate devices9 and wrap- around-gate configurations fabricated jointly by IBM and Harvard University.
The on/off current ratio in a CNT FET is generally high: 104–106, ideal for logic devices. Another important parameter is its transconductance gm—the change in drain–source current Ids divided by the change in the gate voltage Vgs at a constant drain–source voltage. For example, a top-gated FET with a high-dielectric insulator and a CNT diameter of about 1.7 nm can reach a gm of about 30 microsiemens.8 The theoretical limit is close to 155 μS, which leaves a lot of room for improvement.
Another important characteristic of FET operation is what’s known as subthreshold swing, S = dVgs/dlogIds , which describes the voltage needed to produce an order of magnitude change in the current. Conventional FETs require a change in the channel potential of at least 60 mV at 300 K to produce that change, and the minimum subthreshold swing imposes a lower limit on the operating voltage and hence on the power dissipation in conventional FETs. Researchers have achieved that thermodynamic limit in CNT FETs by suppressing SBs in double-gate devices9 or by doping the
www.physicstoday.org
Figure 3. The five-stage ring oscillator pictured in these scanning electron microscope images is composed of 10 FETs built entirely on one 18-μm-long single-walled carbon nanotube. An image of a human hair in the background provides the relative scale of the least-magnified image (circled). Highermagnification images reveal more detail, with the nanotube visible in the upper right. To obtain both p- and n-type FETs on the same CNT, metals with different work functions are chosen as the gates—palladium (Pd) for the p-type FETs and aluminum (Al) for the n-type FETs. The gate oxide is Al2O3, and the ring frequency is 70 MHz at 1 V. (Adapted from
Z. Chen et al., Science 311, 1735, 2006.)
contacts. More important, work at the Watson Research Center has shown that the limit can be exceeded. By taking advantage of band-to-band tunneling, Joerg Appenzeller and colleagues showed that the electron distribution can be filtered to remove high-energy electrons and reduce the subthreshold swing.3
High-speed devices, of course, are a key goal of the whole effort. Consider a typical measure of the frequency response of conventional transistors, the cut-off frequency gm/2πCgs. Using 5 aF as a typical gate–source capacitance Cgs and gm ≈ 10–20 μS for a 100-nm-length CNT FET, we find a cut-off frequency in the range of 1 THz.3 Direct measurement of such high frequencies is difficult because of the mismatch in impedances of CNT FETs and the measurement systems. Indirect measurements on single CNT FETs have shown that their performance is currently limited by the parasitic capacitance of the circuits.
CNTs can also help alleviate the power dissipation problem that limits the scaling of MOSFETs. To dynamically
switch a device takes an energy of ½(Cdev + Cw)V 2, where Cdev and Cw are the device and wiring capacitance contributions,
respectively. A primary advantage of CNT FETs over Si MOSFETs is their much lower capacitance values, roughly 0.05 aF/nm. Careful design, though, has to ensure that parasitic capacitance and Cw, typically 0.2 aF/nm, do not overwhelm Cdev, as they might, for example, when the wires are long. The power dissipation can also be lowered by reducing the supply voltage Vdd (power fVdd2, where f is the operating frequency). However, Vdd cannot be arbitrarily reduced. The performance, τ = 1/f, degrades with decreasing Vdd. As a result, there is an optimum Vdd for a given f.
Integrated circuits
The fabrication of CNT-based devices has advanced beyond single transistors to include logic gates and more complex structures such as ring oscillators.3 The oscillators are composed of an odd number of pairs of p- and n-type FETs made by appropriate doping of the CNTs. Controlled doping in nanoscale devices is difficult, however, and fluctuations in
January 2009 Physics Today 37
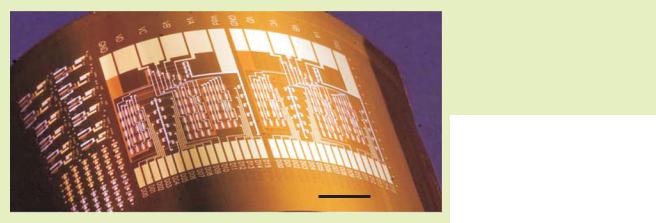
Figure 4. Carbon nanotube thin-film transistors in this optical image of an integrated circuit are bonded to a flexible plastic surface. (Adapted from
Q. Cao et al., ref. 10.)
5 mm
the number and position of the dopants can have a profound effect on device performance.
Fortunately, the ambipolar behavior of an undoped CNT can be used to implement CMOS architecture. A CMOS logic device is formed using a pair of p- and n-type transistors that operate under conditions in which one FET is turned on and the other is off. The ambipolar characteristics of a CNT FET provide a pair of p- and n-type branches. But the leakage branch of one type has a current level comparable to the main branch of the other. Controlling the threshold voltage in an ambipolar device is therefore key to achieving the p- and n- type characteristics suitable for CMOS logic. One can control the threshold voltage by tuning the work function of the metal gate in each FET; the gate work function can be viewed as an extra voltage source added to Vgs. When the metals are properly selected, their different work functions shift the characteristics of p- and n-type branches to generate a distinguishable on state in one CNT FET and an off state in the other.
That approach was employed by IBM’s Zhihong Chen and coworkers to fabricate the most complex electronic structure so far based on a single CNT. Figure 3 shows a scanning electron microscope image of a five-stage ring oscillator in which Pd was chosen as the metal gate for the p-type FET and Al for the n-type FET.
Despite the outstanding performance of single-CNT FETs, their practical implementation faces a number of hurdles. The principal one is the heterogeneity of as-prepared CNT mixtures. Synthetic techniques have emerged in recent years for producing ever-smaller CNT diameter and chirality distributions.
One electronic technology that bypasses the heterogeneity problem to some extent is that of CNT thin-film transistors (TFTs), introduced by Eric Snow and coworkers in 2003.10 A film of randomly oriented CNTs is deposited on an insulator and electrodes are patterned on top of it. Ensemble averaging is expected to correct for the heterogeneity of the film. The presence of metallic CNTs in the mixture poses a problem, however, since they tend to short out the FET channel.
To avoid the problem, the channel is made much longer than the average length of the CNTs so that no single tube can bridge the source–drain gap. Charge transport occurs along “percolation paths,” and switching involves numerous tube–tube junctions. The technique achieves reasonably high on/off current ratios, but at the cost of increasing the channel resistance and consequently producing low drive currents. The carrier mobility is also reduced drastically, although it
38 January 2009 Physics Today
One approach to improving the performance of TFTs is to use purely semiconducting CNTs in a film. Another is to use
highly oriented CNTs, which reduces the number of highresistance tube–tube junctions that are so prevalent in randomly oriented CNTs. Researchers at the Watson Research Center and Northwestern University have recently implemented those improvements in their films, and now both high driving currents (up to milliamps) and fairly high on/off current ratios (roughly 104) can be achieved simultaneously. The mobilities are high for TFTs, but they are still significantly lower than those of single-CNT devices.
Analysis of those results suggests that at least two problems remain. A distribution of tube diameters, and thus bandgaps, still exists in the films. That leads to a spread in the threshold voltage for switching the current in the different tubes. Moreover, high currents require a high CNT density, but closely spaced nanotubes tend to interact and screen the gate field.
Excited states
Studies of the excited states of CNTs have contributed greatly to our understanding of the electronic structure and interactions of CNTs and opened the door to new applications.11 Early on, the electronic excitations of CNTs were discussed, in the context of the single-particle model, as transitions between corresponding pairs of so-called van Hove singularities in the 1D density of states that produce free electron– hole pairs. However, as pointed out by Tsuneya Ando while at the University of Tokyo in 1997, electron–electron interactions should be strong in a confined 1D system and the interactions should form bound states, known as excitons, below the free-particle continuum. First-principle calculations by Steven Louie and coworkers at the University of California, Berkeley, showed that exciton binding energies in nanotubes are unusually large (on the order of an eV). Excitons carry most of the oscillator strength and should, therefore, dominate the absorption spectra (see figure 5).12
The predictions were confirmed by two-photon excitation spectroscopy by Tony Heinz’s group at Columbia University and Christian Thomsen’s group at the Technical University of Berlin.13 The exciton binding energies of semiconducting CNTs depend inversely on their diameter and, unlike the case of bulk semiconductors, also depend on the screening provided by the medium in which the CNTs are embedded.11
Experimental studies of the excited states of nanotubes did not start in earnest until 2002 when Bruce Weisman and coworkers at Rice observed photoluminescence from CNTs wrapped by surfactants and dispersed individually in solu-
www.physicstoday.org
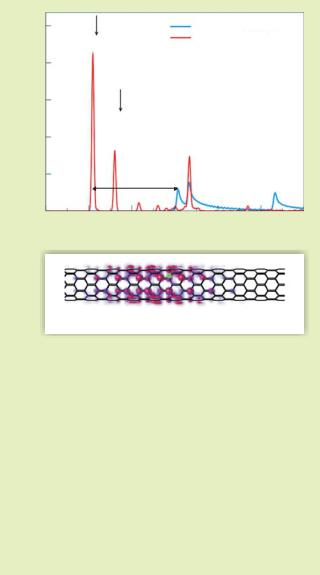
tion.5 Previous attempts were hampered by aggregation effects and the coexistence of metallic and semiconducting CNTs, with the former acting as luminescence quenchers. Once the conditions for luminescence were established, the field grew rapidly. A key application has been the identification of the structure of emitting CNTs. By monitoring the luminescence intensity as a function of laser frequency, researchers can determine the excitation spectra of individual tubes and deduce their structure.5
Following the observation of photoluminescence from CNTs, the decay of their excited states became an active research field. Measured room-temperature fluorescence lifetimes from dispersions of CNTs are in the range of 10–100 ps, although theory predicts CNT radiative lifetimes of 1–10 ns. Reported fluorescence quantum yields, Q, are also low, but there are significant uncertainties in their values.11 However, despite the lack of precise Q values, it is clear that fast nonradiative decay processes operate in CNTs.
Another result of the strong electron–electron interactions in CNTs is that the degeneracy present in the singleparticle states is partially removed, which results in new excitonic states. The transition to the lowest energy state was shown by theory and experiment to be “dark”—that is, dipole forbidden. Trapping in the dark exciton state might be responsible for the observed low yield. However, the energy separation between dark and bright excitons determined by Junichiro Kono and coworkers at Rice is too small to account for the low Q. Recent theoretical work at the Watson Research Center predicts two fast CNT decay channels: multiphonon decay of trapped excitons and a new, phonon-assisted electronic decay pathway.14 The latter is made possible by doping the CNT through charge-transfer interactions with its environment or by external fields.
The photoluminescence statistics of individual CNTs are also interesting. A recent study by Atac Imamoglu and coworkers at ETH Zürich found evidence of photon antibunching, a discovery that may lead to the use of CNTs as single-photon sources and in quantum cryptography.
Electroluminescence
From a photonics-applications perspective, a key finding was that light emission from CNTs can be obtained by passing a current through them.6 As noted previously, CNT FETs tend to be ambipolar, so electron and hole currents can flow simultaneously. When those electrons and holes recombine, a fraction of the recombination events emit a photon and thus give rise to electroluminescence. EL is widely used to produce solid-state light sources such as LEDs.
Conventionally, EL is generated near the interface between a hole-doped and an electron-doped material. But in an ambipolar CNT, doping is unnecessary, which simplifies LED fabrication. Even more interesting, EL from a long CNT does not originate from a fixed point but can actually be translated at will by applying an appropriate bias at the gate;6 the gate bias determines the position where electron–hole recombination takes place in the intrinsic material. The energy E of the EL is the same as that of photoluminescence of the CNT and can thus be tuned by using tubes of different diameters d since EEL is proportional to 1/d.
Another mechanism by which a current can excite EL involves the presence of hot carriers in CNTs. In a unipolar CNT at high bias, the carriers can achieve high enough energies and, because of the strong Coulomb interactions among them, can induce impact excitation and ionization.15 In bulk solids, ionization is the principal process induced, but in the quasi-1D CNTs with high exciton binding energies, neutral
a |
|
|
|
|
|
exp |
|
|
|
|
|
|
|
|
50 |
|
|
|
|
|
|
Without e–h interaction |
|
|
|||
SECTION |
|
|
|
|
E11 |
|
|
|
|
|
|||
|
|
|
|
|
|
|
With e–h interaction |
|
|
||||
|
|
|
A′ |
|
|
|
|
|
|
||||
|
|
|
|
|
|
|
|
|
|
||||
|
|
|
|
|
|
|
|
|
|
|
|
||
CROSS |
|
40 |
|
|
|
|
|
|
|
|
|
|
|
2 |
|
|
|
|
|
|
|
|
|
|
|
|
|
|
/tube) |
30 |
|
|
|
Eexp22 |
|
|
|
|
|
|
|
ABSORPTION |
(nm |
20 |
|
|
|
B′ |
A |
C′ |
C |
|
|
||
|
|
|
|
|
|
|
|
|
|
||||
|
|
|
|
|
|
|
|
|
|
|
|||
|
|
|
|
|
|
|
|
|
|
|
|
||
|
|
10 |
|
|
|
|
|
|
B |
|
|
|
|
|
|
|
|
|
|
|
|
|
|
|
|
||
|
|
0 |
|
|
|
|
|
|
|
|
|
|
|
|
|
|
|
|
|
|
|
|
|
|
|
|
|
|
|
|
|
|
|
|
|
|
|
|
|
|
|
|
|
1.0 |
1.5 |
|
2.0 |
2.5 |
3.0 |
3.5 |
4.0 |
PHOTON ENERGY (eV)
b
+Ψ(re, rh)+2
Figure 5. (a) Ab initio calculations of the absorption spectra of a single-walled carbon nanotube. The electron–hole (e–h) interactions are included in one case (red) but not in the other (blue). The energy and intensity differences between the three lowest interband transitions (A, B, C) and the corresponding exciton states (A’, B’, C’) indicate the pronounced effect of strongly bound e–h pairs in a nanotube. E11 and E22 signify the transition energies experimentally observed using light polarized along the nanotube axis. The energy difference between the first red and blue peaks (shown by the black horizontal arrow) gives the binding energy of the first exciton. (b) A real-space representation of the e–h pair probability distribution, +Ψ(re, rh)+2, as a function of the electron position re when the hole position rh, the green dot, is fixed. (Adapted from ref. 12.)
excitations dominate. Typically, the emission is localized at defect sites, where voltage drops provide enough energy to the carriers to exceed the excitation threshold. More important, one can choose the location of the emission by introducing along the CNT channel a voltage drop—for example, by locally modifying the coupling between the gate and the CNT.15
Electroluminescence from CNTs is broad and nondirectional. But most optoelectronics applications, such as optical interconnections, require a narrow, directional light beam. IBM’s Fengnian Xia and coworkers recently achieved that effect by confining a single CNT in a planar photonic halfwavelength microcavity. In that configuration, the fact that different diameter nanotubes in a sample have slightly different emission energies becomes less important, because the resonant wavelength is determined by the structure of the cavity itself.
Photoconductivity, essentially the reverse of EL, occurs when optical radiation produces free electrons and holes in a material. The process was first observed in CNTs at the Watson Research Center in 2003, but since then many such studies have appeared.11 The same CNT FET device can be used as a transistor, photoswitch, light emitter, or photodetector.
www.physicstoday.org |
January 2009 Physics Today 39 |
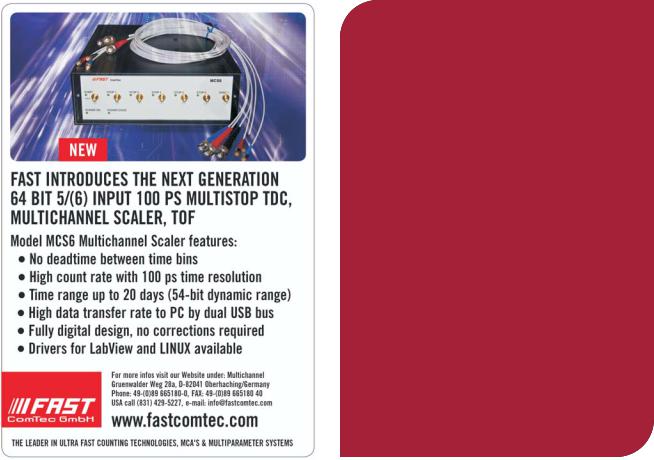
In an open-circuit configuration, internal electric fields can separate electrons and holes and generate a photovoltage. Photovoltage imaging microscopy can provide images of electric fields along CNTs such as those at SBs or charged defects.
On the horizon
With their unique properties, semiconducting nanotubes can form the basis for extremely small, fast, and flexible transistor circuits. CNT devices are molecular devices; therefore, simple and inexpensive self-assembly-based fabrication may be possible. Transistors with chemically modified CNTs are already being used as sensitive and selective chemical and biological sensors. CNT-based light sources and detectors may give rise to intrachip optical communication and singlemolecule spectroscopy. And the excellent electrical conductivity of metallic CNTs may eventually allow the development of electronic systems in which both active devices and passive interconnects are based on CNTs. Continued integration of optics and solid-state devices could set the stage for a unified, CNT-based electronic–optoelectronic technology, and the long spin coherence lengths in CNTs could also lead to quantum interference and spintronic devices.
How soon can we expect to see such developments? The main hurdle lies in the production of large amounts of identical nanostructures. Nanotubes come in many sizes and structures, and there is no reliable way at present to directly produce any single type of CNT needed in a large integrated system. Promising signs suggest that may change. Already, separation of a single type of CNT from mixtures has been successful. And researchers who investigate self-assembly of CNT devices are reporting encouraging results.
The evolution cannot be characterized as an incremental improvement of existing technology as embodied in the scal-
ing of silicon devices. It involves fundamental changes in materials, processing, assembly techniques, and physical principles. The development of CNT technology depends, as does everything else in industry, on cost-benefit analysis and unanticipated breakthroughs. Already, however, the study of CNTs has provided us with unique insights into the physics and chemistry of nanoscale materials.
References
1.S. Iijima, Nature 354, 56 (1991).
2.K. S. Novoselov et al., Nature 438, 197 (2005).
3.A. Jorio, M. S. Dresselhaus, G. Dresselhaus, eds., Carbon Nanotubes: Advanced Topics in the Synthesis, Structure, Properties, and Applications, Springer, New York (2008); Ph. Avouris, Z. Chen, V. Perebeinos, Nat. Nanotechnol. 2, 605 (2007).
4.S. J. Tans, A. R. M. Verschueren, C. Dekker, Nature 393, 49 (1998); R. Martel et al., Appl. Phys. Lett. 73, 2447 (1998).
5.M. J. O’Connell et al., Science 297, 593 (2002); S. M. Bachilo et al., Science 298, 2361 (2002).
6.J. A. Misewich et al., Science 300, 783 (2003); M. Freitag et al., Phys. Rev. Lett. 93, 076803 (2004).
7.R. Martel et al., Phys. Rev. Lett. 87, 256805 (2001); S. Heinze et al.,
Phys. Rev. Lett. 89, 106801 (2002).
8.A. Javey et al., Nature 424, 654 (2003); A. Javey et al., Nat. Mater. 1, 241 (2002).
9.Y.-M. Lin et al., IEEE Trans. Nanotechnol. 4, 481 (2005).
10.J. Novak et al., Appl. Phys. Lett. 83, 4026 (2003); Q. Cao et al., Nature 454, 495 (2008).
11.Ph. Avouris, M. Freitag, V. Perebeinos, Nat. Photonics 2, 341 (2008).
12.C. D. Spataru et al., Phys. Rev. Lett. 92, 077402 (2004).
13.F. Wang et al., Science 308, 838 (2005); J. Maultzch et al., Phys. Rev. B 72, 241402 (2005).
14.V. Perebeinos, Ph. Avouris, Phys. Rev. Lett. 101, 057401 (2008).
15.J. Chen et al., Science 310, 1171 (2005); V. Perebeinos, Ph. Avouris,
Phys. Rev. B 74, 121410 (2006). |
|
UHV SURFACE
SCIENCE
Advanced quadrupole mass spectrometers with UHV specific pulse ion counting detector for:
●photon and electron stimulated desorption
●molecular beam studies
●temperature programmed desorption
9. EPIC systems
fast 100 nanosecond time resolution
high sensitivity positive and negative ions
integration of process data
low profile and X-beam ioniser options
for further details of Hiden Analytical products contact:
info@hideninc.com
www.HidenInc.com
Q u a d r u p o l e s f o r a d v a n c e d s c i e n c e