
- •Contents
- •Preface to the second edition
- •Preface to the first edition
- •1 Special relativity
- •1.2 Definition of an inertial observer in SR
- •1.4 Spacetime diagrams
- •1.6 Invariance of the interval
- •1.8 Particularly important results
- •Time dilation
- •Lorentz contraction
- •Conventions
- •Failure of relativity?
- •1.9 The Lorentz transformation
- •1.11 Paradoxes and physical intuition
- •The problem
- •Brief solution
- •2 Vector analysis in special relativity
- •Transformation of basis vectors
- •Inverse transformations
- •2.3 The four-velocity
- •2.4 The four-momentum
- •Conservation of four-momentum
- •Scalar product of two vectors
- •Four-velocity and acceleration as derivatives
- •Energy and momentum
- •2.7 Photons
- •No four-velocity
- •Four-momentum
- •Zero rest-mass particles
- •3 Tensor analysis in special relativity
- •Components of a tensor
- •General properties
- •Notation for derivatives
- •Components
- •Symmetries
- •Circular reasoning?
- •Mixed components of metric
- •Metric and nonmetric vector algebras
- •3.10 Exercises
- •4 Perfect fluids in special relativity
- •The number density n
- •The flux across a surface
- •Number density as a timelike flux
- •The flux across the surface
- •4.4 Dust again: the stress–energy tensor
- •Energy density
- •4.5 General fluids
- •Definition of macroscopic quantities
- •First law of thermodynamics
- •The general stress–energy tensor
- •The spatial components of T, T ij
- •Conservation of energy–momentum
- •Conservation of particles
- •No heat conduction
- •No viscosity
- •Form of T
- •The conservation laws
- •4.8 Gauss’ law
- •4.10 Exercises
- •5 Preface to curvature
- •The gravitational redshift experiment
- •Nonexistence of a Lorentz frame at rest on Earth
- •The principle of equivalence
- •The redshift experiment again
- •Local inertial frames
- •Tidal forces
- •The role of curvature
- •Metric tensor
- •5.3 Tensor calculus in polar coordinates
- •Derivatives of basis vectors
- •Derivatives of general vectors
- •The covariant derivative
- •Divergence and Laplacian
- •5.4 Christoffel symbols and the metric
- •Calculating the Christoffel symbols from the metric
- •5.5 Noncoordinate bases
- •Polar coordinate basis
- •Polar unit basis
- •General remarks on noncoordinate bases
- •Noncoordinate bases in this book
- •5.8 Exercises
- •6 Curved manifolds
- •Differential structure
- •Proof of the local-flatness theorem
- •Geodesics
- •6.5 The curvature tensor
- •Geodesic deviation
- •The Ricci tensor
- •The Einstein tensor
- •6.7 Curvature in perspective
- •7 Physics in a curved spacetime
- •7.2 Physics in slightly curved spacetimes
- •7.3 Curved intuition
- •7.6 Exercises
- •8 The Einstein field equations
- •Geometrized units
- •8.2 Einstein’s equations
- •8.3 Einstein’s equations for weak gravitational fields
- •Nearly Lorentz coordinate systems
- •Gauge transformations
- •Riemann tensor
- •Weak-field Einstein equations
- •Newtonian limit
- •The far field of stationary relativistic sources
- •Definition of the mass of a relativistic body
- •8.5 Further reading
- •9 Gravitational radiation
- •The effect of waves on free particles
- •Measuring the stretching of space
- •Polarization of gravitational waves
- •An exact plane wave
- •9.2 The detection of gravitational waves
- •General considerations
- •Measuring distances with light
- •Beam detectors
- •Interferometer observations
- •9.3 The generation of gravitational waves
- •Simple estimates
- •Slow motion wave generation
- •Exact solution of the wave equation
- •Preview
- •Energy lost by a radiating system
- •Overview
- •Binary systems
- •Spinning neutron stars
- •9.6 Further reading
- •10 Spherical solutions for stars
- •The metric
- •Physical interpretation of metric terms
- •The Einstein tensor
- •Equation of state
- •Equations of motion
- •Einstein equations
- •Schwarzschild metric
- •Generality of the metric
- •10.5 The interior structure of the star
- •The structure of Newtonian stars
- •Buchdahl’s interior solution
- •10.7 Realistic stars and gravitational collapse
- •Buchdahl’s theorem
- •Quantum mechanical pressure
- •White dwarfs
- •Neutron stars
- •10.9 Exercises
- •11 Schwarzschild geometry and black holes
- •Black holes in Newtonian gravity
- •Conserved quantities
- •Perihelion shift
- •Post-Newtonian gravity
- •Gravitational deflection of light
- •Gravitational lensing
- •Coordinate singularities
- •Inside r = 2M
- •Coordinate systems
- •Kruskal–Szekeres coordinates
- •Formation of black holes in general
- •General properties of black holes
- •Kerr black hole
- •Dragging of inertial frames
- •Ergoregion
- •The Kerr horizon
- •Equatorial photon motion in the Kerr metric
- •The Penrose process
- •Supermassive black holes
- •Dynamical black holes
- •11.6 Further reading
- •12 Cosmology
- •The universe in the large
- •The cosmological arena
- •12.2 Cosmological kinematics: observing the expanding universe
- •Homogeneity and isotropy of the universe
- •Models of the universe: the cosmological principle
- •Cosmological metrics
- •Cosmological redshift as a distance measure
- •The universe is accelerating!
- •12.3 Cosmological dynamics: understanding the expanding universe
- •Critical density and the parameters of our universe
- •12.4 Physical cosmology: the evolution of the universe we observe
- •Dark matter and galaxy formation: the universe after decoupling
- •The early universe: fundamental physics meets cosmology
- •12.5 Further reading
- •Appendix A Summary of linear algebra
- •Vector space
- •References
- •Index
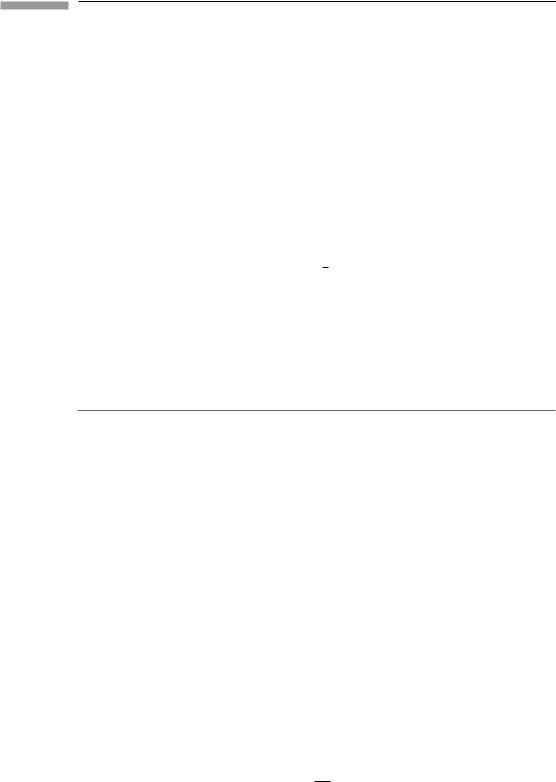
358 |
Cosmology |
So far we have restricted our attention to the case of a positive cosmological constant. While this seems to be the most relevant to the evolution of our universe, cosmologies with negative cosmological constant are also interesting. We leave their exploration to the exercises.
Einstein introduced the cosmological constant in order to allow his equations to have a
˙ =
static solution, R 0. He did not know about the Hubble flow at the time, and he followed the standard assumption of astronomers of his day that the universe was static. Even in the framework of Newtonian gravity, this would have presented problems, but no-one seems to have tried to find a solution until Einstein addressed the issue within general relativity.
˙ =
We have to do more than just set R 0 in Eq. (12.54); we have to guarantee that the
˙
solution is an equilibrium one, that the dynamics won’t change R, i.e. that the universe is at a minimum or maximum of the ‘potential’ we discussed earlier. We show in Exer. 20, § 12.6 that the static solution requires
1
ρ = 2 ρ0.
For Einstein’s static solution, the dark energy density has to be exactly half of the matter energy density. We shall see below that in our universe the measured value of the dark energy density is about twice that of the matter energy density, so we are near to but not exactly at Einstein’s static solution.
Critical density and the parameters of our universe
If we divide Eq. (12.54) by 4π R2/3, we obtain a version that is instructive for discussions of the physics of the universe:
3H2 |
= − |
3k |
+ ρm + ρ , |
(12.58) |
|
|
8π |
8π R2 |
|||
|
|
|
|
|
|
˙
where we have substituted the Hubble parameter H for R/R. Since the last two terms on the right are energy densities, it is useful to interpret the other terms in that way. Thus, the Hubble expansion has associated with it an energy density ρH = 3H2/8π , and the spatial curvature parameter contributes an effective energy density ρk = −3k/8π R2. This equation becomes
ρH = ρk + ρm + ρ .
Now, if in the universe today the ‘physical’ energy density ρm + ρ is less than the Hubble energy density ρH , then (as we have seen before), the curvature energy density must be positive, the curvature parameter k must be negative, and the universe has hyperbolic hypersurfaces. Conversely, if the physical energy density is larger than the Hubble energy density, the universe will be the closed model. The Hubble energy density is therefore a threshold, and we call it the critical energy density ρc:
ρc = 3 (12.59) 8π
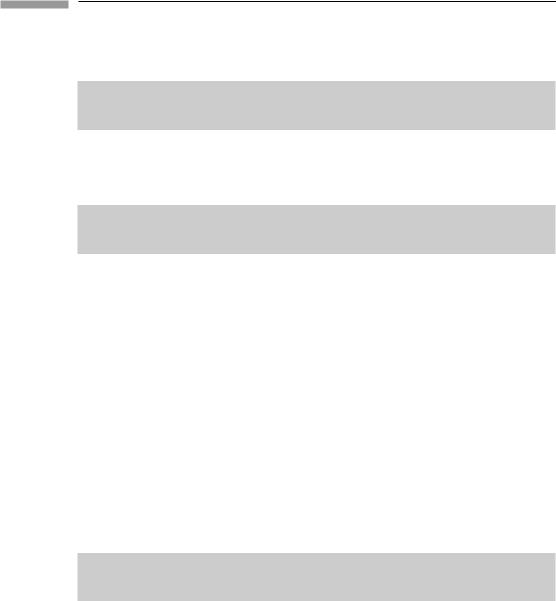
359 |
12.3 Cosmological dynamics: understanding the expanding universe |
The ratio of any energy density to the critical is called with an appropriate subscript. Thus, we can divide the earlier energy-density equation, evaluated at the present time, by ρc to get
1 = k + m + . |
(12.60) |
These are the quantities used to label the curves in Fig. 12.3. The data from supernovae, the cosmic microwave background, and studies of the evolution of galaxy clusters (below) all suggest that our universe at present has
= 0.7, m = 0.3, k = 0. |
(12.61) |
These mean that we live in a flat universe, dominated by a positive cosmological constant.
What size do these numbers have? It is conventional among astronomers to normalize the Hubble constant H0 to the value 100 km s−1 Mpc−1 by introducing the scaled Hubble constant h (nothing to do with gravitational wave amplitudes!):
h = H0/100 km s−1 Mpc−1. |
(12.62) |
The best value today is h = 0.71. Using this, the critical energy density is
ρc = 1.88 × 10−26h2kg m−3 = 9.5 × 10−27kg m−3.
As we have noted, the matter energy density is about 0.3 times this, and this is much more than astronomers can account for by counting stars and galaxies. In fact, studies of the formation of elements in the early universe (below) tell us that the density of baryonic matter (normal matter made of protons, neutrons, and electrons) has b = 0.04. So most of the matter in the universe is non-baryonic, does not emit light, and can be studied astronomically only indirectly, through its gravitational effects. This is called dark matter. So we can split m into its components:
m = b + d, b = 0.04, d = 0.26. |
(12.63) |
We will return in § 12.4 below to a discussion of the nature and distribution of the dark matter. The values in Eqs. (12.61) and (12.63) are commonly referred to as the concordance cosmology.
The variety of possible cosmological evolutions and the data are captured in the diagram in Fig. 12.4. The evidence is getting rather strong that the dark energy is present, and even dominant. That raises new, important questions. The deepest is, where in physics does this energy come from? We will mention below some of the speculations, but at present there is simply no good theory for it. In such a situation, better data might help. For example, astronomers could try to determine if the dark energy density really is constant in time (as it would be if it comes from a cosmological constant) or variable, which would indicate

360
Figure 12.4
Cosmology
Supernova cosmology project
3
No big bang
Knop et al. (2003)
Spergel et al. (2003)
Allen et al. (2202)
2
Supernovae
1
M |
|
|
|
|
Ω |
|
|
|
|
|
CMB |
|
|
|
|
|
Expands |
forever |
|
0 |
|
ally |
|
|
|
|
|
||
|
recollapses |
eventu |
|
|
|
|
|
|
|
|
Clusters |
|
|
|
|
|
Closed |
|
|
|
|
Flat |
|
|
–1 |
|
Open |
|
|
|
|
|
|
|
0 |
1 |
2 |
|
3 |
|
|
ΩM |
|
|
In the m v. plane one sees the variety of possible cosmological models, their histories and futures. The constraints from studies of supernovae (Knop et al. 2003), the cosmic microwave background radiation (Spergel et al. 2003), and galaxy clustering (Allen et al. 2002) are consistent with one another and all overlap in a small region of parameter space centered on= 0.7 and m = 0.3. This means that k = 0 to within the errors. Figure courtesy the Supernova Cosmology Project.
that it comes from some physical field masquerading as a cosmological constant. As of this writing, new space and ground-based observing programs are being planned, so that in another decade we might have a new generation of ultra-precise measurements of the dark energy.
From the point of view of general relativity, one of the most intriguing ways of studying the dark energy is with the LISA gravitational wave detector. As mentioned in § 9.5, LISA will be able to observe coalescences of black holes at high redshifts and measure their distances. What will be measured from the signal is the luminosity distance dL to the binary, since it is based on an inference of the luminosity of the system in gravitational waves from the information contained in the signal. This measurement can be made with great accuracy, perhaps with errors at the few percent level. To do cosmography, we have to combine these luminosity distance measures with redshifts, and that will not be easy: black hole coalescences do not give off any electromagnetic radiation directly, so it will not be easy to identify the galaxy in which the event has occurred. But the galaxies hosting the mergers will not be normal galaxies, and the merger event might be accompanied by other