
Enzymes (Second Edition)
.pdf370 COOPERATIVITY IN ENZYME CATALYSIS
0 moles of bound O ; under equilibrium conditions, no significant population of hemoglobin molecules exist with intermediate (i.e., 2 or 3) stoichiometries of
O binding.
The crystal structures of oxy- (with four O molecules bound) and deoxy- (with no O bound) hemoglobin provide a clear structural basis for this cooperativity. We know from Chapter 3 that hemoglobin can adopt two distinct quaternary structures; these are referred to as the R (for relaxed) and T (for tense) states (see Section 12.2). The differences between the R and T quaternary structures are relative rotations of two of the subunits, as described in Figure 3.18. These changes in quaternary structure are mediated by changes in intersubunit hydrogen bonding at the subunit interfaces. The crystal structures of oxyand deoxyhemoglobin reveal that loss of oxygen at the heme of one subunit induces a change in the strength of the iron—histidine bond that occupies the fifth coordination site on the heme iron. This change in bond strength results in a puckering of the porphyrin macrocycle and a displacement of position for the coordinated histidine (Figure 12.2). The coordinated histidine is located in a segment of -helical secondary structure in the
hemoglobin subunit, and the motion of the histidine in response to O binding
or release results in a propagated motion of the entire helix. Ultimately, this propagated motion produces alterations of the intersubunit hydrogen-bonding pattern at the / subunit interface that acts as a quaternary structure ‘‘switch.’’ The accompanying movements of the other subunits leads to alterations of the oxygen affinities for their associated heme cofactors.
The availability of detailed structural information for both the oxy and deoxy structures of hemoglobin has made this molecule the classic model of cooperativity in proteins, illustrating how distant binding sites can interact to control the overall affinity for a single ligand. Likewise, the structural information available for the Trp repressor protein has made this molecule an excellent example of allosteric regulation in biology. As its name implies, the Trp repressor protein acts to inhibit the function of the Trp operon, a segment of DNA that is ultimately responsible for the synthesis of the amino acid tryptophan. The protein accomplishes this task by binding within the major groove of the DNA in its tryptophan-bound form and, when not bound by tryptophan, releasing the DNA. The activity of the Trp repressor is an example of a negative feedback loop, in which the synthesis of an essential molecule of the cell is controlled by the concentration of that molecule itself. At low tryptophan concentrations, the synthesis of tryptophan is required by the cell. Under these conditions the Trp operon must be functional, and thus the Trp repressor must not bind to the DNA.
The crystal structures of the tryptophan-depleted protein shows that the-helical segments of the protein are arranged in a way that precludes effective DNA binding (Figure 12.3A). Thus, when the tryptophan concentration is low, the protein is found in a conformation that does not allow for DNA binding, and the operon is functional, leading to tryptophan synthesis. When the tryptophan concentration in the cell exceeds some critical concentration,
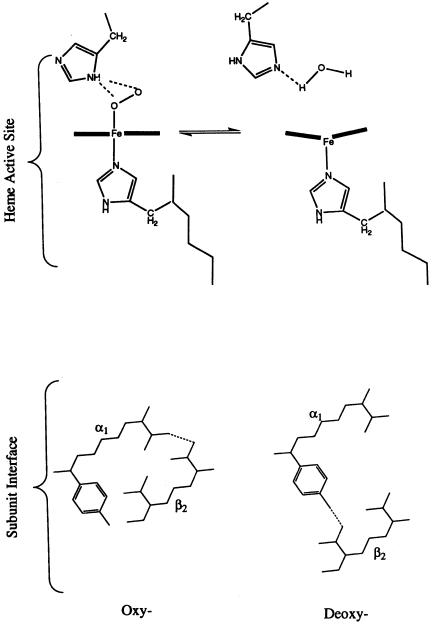
HISTORIC EXAMPLES OF COOPERATIVITY AND ALLOSTERY IN PROTEINS |
371 |
Figure 12.2 Changes in structure of the active site heme that accompany O2 binding to hemoglobin, and the associated changes in protein structure at the 1/ 2 subunit interface.
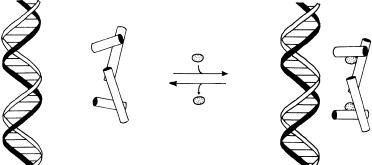
372 COOPERATIVITY IN ENZYME CATALYSIS
(A) |
(B) |
Figure 12.3 Cartoons of the interactions of the Trp repressor protein with Trp operon DNA in the absence (A) and presence (B) of bound tryptophan. This tryptophan-binding-induced conformational transition is the basis for the negative feedback regulation of tryptophan synthesis.
however, the Trp repressor binds tryptophan and, as a result, changes its conformation. The tryptophan-replete form of the protein now has an -helical arrangement in which two helices are positioned for effective binding to the Trp operon, via interactions between the helices and the double-stranded DNA helical structure (Figure 12.3B). When the Trp repressor binds to the operon, it effectively shuts down the action of this DNA, thus leading to inhibition of further tryptophan synthesis. This simple method of conformationally controlling the activity of the Trp repressor, by binding of tryptophan, provides an elegant mechanismfor the metabolic control of the production of an essential amino acid.
Again, we have used hemoglobin and the Trp repressor to illustrate the concepts of cooperativity and allosteric control in structural terms because of the wealth of structural information available for these two proteins. The reader should be aware, however, that the same mechanisms are common in enzymatic systems as well. Numerous examples of cooperativity and allosteric control of enzymatic activity can be found in biology, and these control mechanisms serve vital metabolic roles. For example, many enzymes involved in de novo biosynthetic cascades display the phenomenon of feedback inhibition. Here a metabolite that is the ultimate or penultimate product of the cascade will act as a heterotropic inhibitor of one of the enzymes that occurs early in the biosynthetic cascade, much as tryptophan controls its own rate of synthesis by binding to the Trp repressor.
One of the first examples of this phenomenon came from studies of threonine deaminase from the bacterium E. coli. Abelson (1954) observed that addition of isoleucine to cultures of the bacterium inhibited the further biosynthesis of isoleucine. Later workers showed that this effect is due to specific inhibition by isoleucine of threonine deaminase, the first enzyme in the biosynthetic route to isoleucine. Further studies with purified threonine
MODELS OF ALLOSTERIC BEHAVIOR |
373 |
deaminase revealed that the substrate threonine and the inhibitor isoleucine bind to the enzyme at different, nonidentical sites; thus isoleucine is an example of a heterotropic allosteric inhibitor of threonine deaminase.
Another classic example of feedback inhibition comes from aspartate carbamoyltransferase. This enzyme catalyzes the formation of carbamoylaspartate from aspartate and carbamoylphosphate, which is the first step in the de novo biosynthesis of pyrimidines. The enzyme shows a sigmoidal dependence of reaction rate on the concentration of the substrate, aspartate, demonstrating cooperativity between the active sites of this oligomeric enzyme. Additionally, the enzyme is inhibited by the pyrimidine analogues cytidine, cytidine 5-phosphate, and cytosine triphosphate (CTP), and is activated by adenosine triphosphate (ATP). Structural studies also have revealed that the substrate binding sites and the CTP inhibitory binding sites are separate and distinct; binding of CTP at its exclusive site, however, influences the affinity of the active site for aspartate, via heterotropic allosteric regulation.
Threonine deaminase and aspartate carbamoyltransferase are examples of what is now known to be a ubiquitous means of metabolic control, namely, feedback inhibition. To understand fully this important biological control mechanism requires a theoretical framework for describing how distant sites within an enzyme can interact to affect one another’s affinity for similar or different ligands. We now turn to a brief description of two such theoretical frameworks that have proved useful in the study of allosteric enzymes.
12.2 MODELS OF ALLOSTERIC BEHAVIOR
When an oligomeric enzyme contains multiple substrate binding sites that all behave identically (i.e., display the same K and k for substrate) and independently, the velocity equation can be shown to be identical to that for a single active site enzyme (Segel, 1975). Regardless of whether the binding sites interact, an oligomeric enzyme will have different distributions of ligand occupancy among its different subunits at different levels of substrate saturation. For instance, six possible combinations of subunit occupancies can be envisaged for a tetrameric enzyme with two substrate molecules bound. This is illustrated for the example of a tetrameric enzyme in Figure 12.4.
When the ligand binding sites of an oligomeric enzyme interact cooperatively, we need to modify the existing kinetic equations to account for this intersite interaction. Two theoretical models have been put forth to explain allostery in enzymes and other ligand binding proteins. The first, the simple sequential interaction model, proposed by Koshland and coworkers (Koshland et al., 1966), is in some ways an extension of the induced-fit model introduced in Chapter 6. The second, the concerted transition or symmetry model, is based on the work of Monod, Wyman, and Changeux (1965) and has been widely applied to proteins such as hemoglobin, to explain ligand binding cooperativity.

374 COOPERATIVITY IN ENZYME CATALYSIS
Figure 12.4 Schematic illustration of the number of possible forms of ligand binding to an enzyme that is a homotetramer: open squares, subunits with empty binding site; shaded squares with S, subunits to which a molecule of substrate has bound to the active site.
The simple sequential interaction model assumes that a large conformational change attends each ligand binding event at one of the enzyme active sites. It is this conformational transition that affects the affinity of the enzyme for the next ligand molecule. Let us consider the simplest case of an allosteric enzyme with two substrate binding sites that display positive cooperativity. The equilibria involved in substrate binding and their associated equilibrium constants are schematically illustrated in Figure 12.5 (Segel, 1975). The dissociation constant for the first substrate molecule is give by K . When one of the substrate binding sites is occupied, however, the dissociation constant for the second site is modified by the factor a, which for positive cooperativity has a value less than 1. The overall velocity equation for such an enzyme is given by:
|
|
[S] |
|
[S] |
|
||||||
V |
|
|
|
|
|
|
|
||||
K |
aK |
(12.1) |
|||||||||
v |
|
|
|
|
|
|
|
||||
1 |
2[S] |
|
[S] |
||||||||
|
|
|
|||||||||
|
|
|
|
|
|
|
|
||||
|
K |
|
aK |
|
|||||||
|
|
|
|
|
|
|
|
|
Now let us extend the model to a tetrameric enzyme (Figure 12.6). In this case the binding of the first substrate molecule modifies the dissociation constant of all three other binding sites by the factor a. If a second substrate molecule now binds, the two remaining vacant binding sites will have their dissociation constants modified further by the factor b, and their dissociation
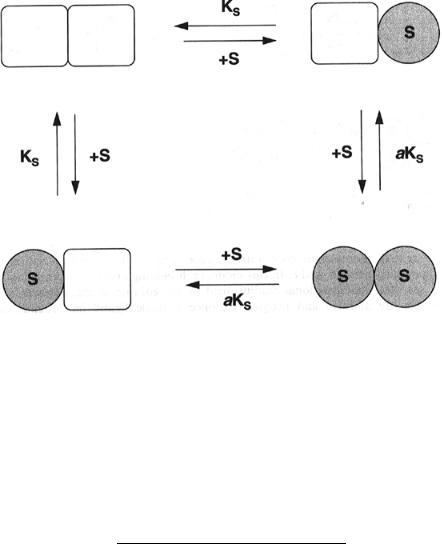
MODELS OF ALLOSTERIC BEHAVIOR |
375 |
Figure 12.5 Schematic representation of the equilibria involved in substrate binding to a homodimeric enzyme where substrate binding is accompanied by a conformational transition of the subunit to which it binds, according to the model of Koshland et al. (1966).
constants will be abK . When a third substrate molecule binds, the final empty binding site will be modified still further by the factor c, and the dissociation constant will be abcK . Taking into account all these factors, and the occupancy weighing factors from Figure 12.4, we can write overall velocity of the enzymatic reaction as follows:
|
|
|
[S] |
|
3[S] |
|
|
3[S] |
|
|
|
[S] |
|
|||||||||
|
V |
|
|
|
|
|
|
|
|
|
|
|
|
|
|
|
|
|||||
K |
|
|
|
aK |
a bK |
a b cK |
(12.2) |
|||||||||||||||
v |
|
|
|
|
|
|
|
|
|
|
|
|
|
|
|
|
||||||
1 |
4[S] |
|
6[S] |
|
4[S] |
|
|
[S] |
||||||||||||||
|
|
|
||||||||||||||||||||
|
|
|
|
|
|
|
|
|
|
|
|
|
||||||||||
|
K |
|
|
|
aK |
|
a bK |
a b cK |
|
|||||||||||||
|
|
|
|
|
|
|
|
|
|
|
|
|
|
|
|
|
||||||
Equation 12.2 is a velocity |
equation |
|
that can |
account for |
either positive |
or negative homotropic cooperativity, depending on the numerical values of the coefficients a, b, and c. In this model each binding event is associated with a separate conformational readjustment of the enzyme. Since, however, the effects are additive and progressive, once a single ligand has bound, the subsequent steps are strongly favored.
The second model for homotrophic cooperativity is the concerted transition or symmetry model, which is also known as the MWC model in honor of its
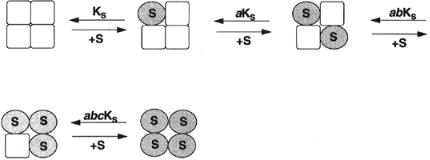
376 COOPERATIVITY IN ENZYME CATALYSIS
Figure 12.6 Extension of the Koshland model, from Figure 12.5, to a tetrameric enzyme.
original proponents: Monod, Wyman, and Changeux. This model assumes that allosteric enzymes are oligomers made up of identical minimal units (subunits or ‘‘protomers’’) arranged symmetrically with respect to one another and that each unit contains a single ligand binding site. The overall oligomer can exist in either of two conformational states, reflecting either a change in quaternary structure or tertiary structure changes within the individual protomer units, and these two conformations are in equilibrium. Another feature of the MWC model is that the transition between the two conformational states occurs with a retention of symmetry. For this to be so, all the protomer units must change in concert—one cannot have an oligomer in a mixed conformational state (i.e., some protomers in one conformation and some in the other).
Hence, in contrast to the Koshland model, in the MWC model the transition between the two conformational states is highly concerted, and there are no hybrid or intermediate states involved. The affinity of the ligand binding site on a protomer depends on the conformational state of that protomer unit. In other words, the ligand of interest will bind preferentially to one of the two conformational states of the protomer. Thus, binding of a ligand to one binding site will shift the equilibrium between the conformational states in favor of the preferred ligand binding conformation. Since the protomeric units of the oligomer shift conformation in concert, ligand binding to one site has the effect of switching all the ligand binding sites to the higher affinity form. Thus the MWC model explains strong positive cooperativity in terms of the observation that occupancy at a single ligand binding site induces all the other binding sites of the oligomeric protein to take on their high affinity conformation.
The original MWC model assumes that the conformational state with low ligand affinity is a strained structure and that the strain is relieved by ligand binding and the associated conformational transition. For this reason, the state of low binding affinity is often referred to as the ‘‘T’’ state (for tense), and the high affinity conformation is referred to as the ‘‘R’’ state (for relaxed). While the transitions between these states are concerted, as described, for bookkeep-
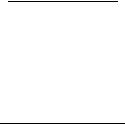
MODELS OF ALLOSTERIC BEHAVIOR |
377 |
ing purposes diagrams of the MWC model designate different ligand occupancy states of the two conformations as R and T , where x indicates the number of ligand bound to the oligomer. Therefore, a tetrameric enzyme could in principle occur in states R through R , and T through T . The states R and T thus refer to the two conformational states with no ligands bound to the enzyme. The equilibrium constant between these two ‘‘empty’’ states, the allosteric constant, is symbolized by L :
L |
[T ] |
|
(12.3) |
[R ] |
|||
|
|
|
This dissociation constant of a binding site for ligand, S, on a protomer in the T state is termed K , and for the protomer in the R state this dissociation constant is K . The ratio K /K is referred to as the nonexclusive binding coefficient and is symbolized by c. Both L and c influence the degree of cooperativity displayed by the enzyme. As L becomes larger, the velocity curve for the enzymatic reaction displays greater sigmoidal character, because the R —T equilibrium favors the T state more. As c increases, the affinity of the T state for ligand increases relative to the R state. Hence, high cooperativity is associated with small values of c.
The simplest example of the MWC model is that for a dimeric enzyme in which the T state is assumed to have no affinity at all for the substrate (i.e., c 0). Figure 12.7 schematically represents the equilibria involved in such a system, where substrate binds exclusively to the R state of the dimer. (Since only the R state has a noninfinite substrate dissociation constant here, we shall use the symbol K in place of K for this system.) The velocity equation for such a system, which can be derived from a rapid equilibrium set of assumptions, yields the following functional form:
|
[S] |
1 |
[S] |
|
|||||||
|
V |
|
|
|
|
||||||
|
K |
|
|
K |
|||||||
v |
|
|
|
|
|
|
|
|
|
(12.4) |
|
|
|
|
|
[S] |
|
||||||
|
|
|
|
|
|
|
|
||||
|
L 1 |
|
|
|
|
|
|||||
|
K |
|
|
|
|||||||
|
|
|
|
|
|
|
|
|
|
|
For oligomers larger than dimers, a generalized form of Equation 12.4 can be derived (Segel, 1975):
|
|
[S] |
|
|
[S] |
|
|
|||
|
V |
|
|
1 |
|
|
|
|
||
|
K |
K |
(12.5) |
|||||||
v |
|
|
|
|
|
|
|
|||
|
|
[S] |
|
|||||||
|
|
|
|
|
|
|||||
|
L 1 |
|
|
|
|
|||||
|
K |
|
|
where h is the total number of ligand binding sites on the oligomeric enzyme. The MWC model provides a useful framework for understanding positive homotropic cooperativity, and it can be modified to account for heterotropic cooperativity as well (Segel, 1975; Perutz, 1990). This model cannot, however,
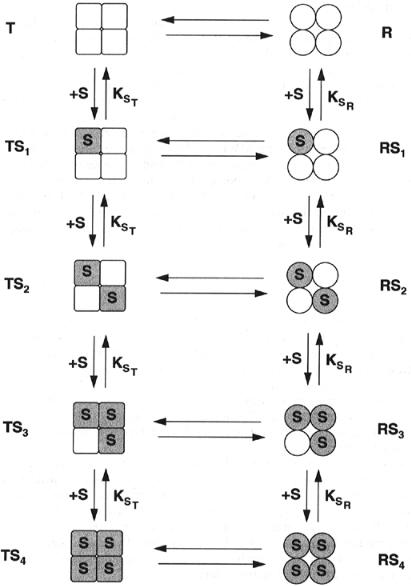
378 COOPERATIVITY IN ENZYME CATALYSIS
Figure 12.7 Schematic representation of the equilibria involved in the binding of substrates to a tetrameric enzyme according to the model of Monod, Wyman, and Changeux (1965). See text for further details.

EFFECTS OF COOPERATIVITY ON VELOCITY CURVES |
379 |
account for the phenomenon of negative homotrophic cooperativity. When negative cooperativity is encountered, it is usually explained in terms of the Koshland sequential interaction model.
12.3 EFFECTS OF COOPERATIVITY ON VELOCITY CURVES
Referring back to the Koshland simple sequential interaction model, we can state that if the cooperativity is large, the concentrations of species with at least one substrate binding site unsaturated will be very small at any concentration of substrate greater than K . In the case of a tetrameric enzyme, for example, under these conditions Equation 12.2 reduces to the much simpler equation:
v V [S]
K [S]
where K a b cK . Equation 12.6 is a specific case enzymes) of the more general simple equation:
v V [S]
K [S]
(12.6)
(i.e., for tetrameric
(12.7)
in which h is the total number of substrate binding sites on the oligomeric enzyme molecule and K is a constant that relates to the individual interaction coefficients a through h, and the intrinsic dissociation constant K . Note that in the absence of cooperativity, and when h 1, Equation 12.6 reduces to an equation reminiscent of the Henri—Michaelis—Menten equation from Chapter 5. When cooperativity occurs, however, the constant K no longer relates to the concentration of substrate required for the attainment of half maximal velocity.
Equation 12.7 is known as the Hill equation, and the coefficient h is referred to as the Hill constant. This simple form of this equation can be readily used to fit experimental data to enzyme velocity curves, as introduced in Chapter 5 (see Figure 5.15). When the degree of cooperativity is moderate, however, contributions from intermediate occupancy species (i.e., number of bound substrate molecules h) may contribute to the overall reaction. In these cases, the experimental data are often still well modeled by Equation 12.7, although the empirically determined value of h will no longer reflect the total number of binding sites on the enzyme, and may not in fact be an integer. In this situation the experimentally determined coefficient is referred to as an apparent h value (sometimes given the symbol h ). The next integer value above the apparent h value is considered to represent the minimum number of binding sites on the oligomeric enzyme.
For example, suppose that the experimentally determined value of h is 1.65. This could be viewed as representing and enzyme with 1.65 highly cooperative substrate binding sites, but of course this makes no physical sense. Instead we