
Engineering Biology for Drugs and Fuels
.pdf
Engineering Biology for Drugs and Fuels1
JAY D. KEASLING
Professor of Chemical Engineering and Bioengineering
University of California, Berkeley
Associate Laboratory Director for Biosciences
Lawrence Berkeley National Laboratory
I’M GOING TO TALK about plug-and-play biology. And I’m going to first introduce it by talking about a problem, a problem many of you may know about. It’s malaria. It actually affects about a quarter of the world’s population; 2.4 billion people are at risk of get-
ting malaria at any one time; between 300 and 500 million people have the disease, and every year between one and three million people die of the disease. Ninety percent are children under the age of five.
And of those countries most affected by malaria, economists have noted that it reduces their GDP by roughly half. So it’s a hugely debilitating disease. The drugs that are currently available are based largely on quinine. Quinine has been around for four hundred years; wars were fought for access to quinine. And it’s been so widely used and abused that Plasmodium, which causes malaria, has grown resistant to it. So, widely available, inexpensive, and no longer effective.
This is artemisinin (fig. 1). It comes from this plant Artemisia annua and it’s a miracle cure, really, for malaria. It’s been around for literally centuries. It was discovered in about 168 BC by the Chinese. There are some writings about its use, first for treating hemorrhoids and later for treating fevers. It was largely forgotten until the 1960s, when the Chinese were fighting in Vietnam and they needed a cure for the severe malaria there. They went back to their literature; they purified the active ingredient, and the rest is history.
Now, here’s how we get artemisinin. It’s actually produced by this plant Artemisia annua in sacs called trichomes on the surface of the leaves. So there are basal cells in these trichomes and they push this oil, this artemisinin, up into these bags, and when you rub the leaf, you actually get it off.
1 Read 13 November 2009. This paper was presented with Ian T. Baldwin’s paper “Training a New Generation of Biologists: The Genome-Enabled Field Biologists,” which appeared in the June 2012 issue of the Proceedings, on pp. 205–14.
PROCEEDINGS OF THE AMERICAN PHILOSOPHICAL SOCIETY VOL. 156, NO. 3, SEPTEMBER 2012
[283]

284 |
jay d. keasling |
Now we get artemisinin from farmers who grow plants. There are a lot of plantations, primarily in Southeast Asia, where they grow the plant. They then purify it, from the plant; they purify it using the only solvent they have available—gasoline. There’s a big problem. A few years ago when they were using leaded gasoline, the lead was contaminating the artemisinin supply, and then that’s sold to the farmer for conversion into the various derivatives that are currently in use for treating malaria. Artesanate looks like it’s going to be the best derivative of artemisinin because it’s water soluble and it can be formulated in IVs.
In 2005 the World Health Organization recommended artemisinin combination therapies as the drug of choice for treating malaria. These bars here (fig. 2) show artemisinin combination therapies delivered over that period. You can see there’s a huge ramp-up primarily around 2006 and 2007. It takes about eighteen months to two years to get seeds in the field and artemisinin delivered to the farmers, so you need a very long lag time.
Now here’s where we project the demand will go. This is largely due to the Gates Foundation, the Clinton Global Fund, and the World Bank’s making these drugs available. So there’s going to be a huge demand for them. We’re going to need on the order of 300 million treatments every year for malaria.
Now when artemisinin was recommended as the drug of choice for treating malaria in 2005, the price spiked. This is one of the severe
Figure 2. Supply of artemisinin and scenarios of need. Source: Boston Consulting Group.
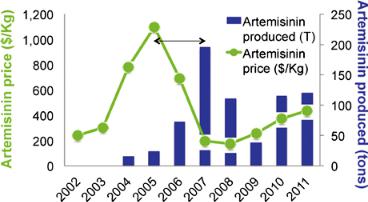
engineering biology |
285 |
Figure 3. Swings in the price of artemisinin and availability. Source: Boston Consulting Group.
problems with artemisinin: the price fluctuates while there is an open market.
So the price spiked and farmers started planting it like crazy, and that is shown right here (fig. 3). By 2007 there was a huge over-supply of artemisinin. What happened? They stopped planting it. The price of food went up, and they are no longer planting artemisinin. You can see that the prices are sitting down here because we have this over-supply, but the problem is that we’re living on that over-supply from 2007.
And guess what? Next year that supply runs out. And in fact we have a deficit. And if you look forward, there’s going to be a huge under-supply by about 250 million treatments, which means the prices are going to go back up. So we’re going to have fluctuations.
We decided that there was a better way to produce artemisinin. We could actually engineer a microbe to be a chemical factory and produce it from something inexpensive like a sugar supply.
Here’s the process diagram (fig. 4). We’re going to engineer a microbe (that’s shown here by the oblong symbol) to produce artemisinin acid—not artemisinin itself, but artemisinin acid. Artemisinin is toxic to Plasmodium, which causes malaria; it’s also toxic to the yeast and E. coli that we might want to use to produce this drug. So we produce the immediate precursor to that drug and then use some standard chemistry to turn that into artemisinin. Then pharma can turn it into the various derivatives that are currently in use. But we need this microbe that’s going to produce the artemisinin acid.
So we start with a chassis. Now when I talk about a chassis, I talk about something that’s similar to the box that harbors all the devices that are now in our computer. For us the chassis, either E. coli or yeast,

286 |
jay d. keasling |
Figure 4. Process diagram for semi-synthetic production of artemisinin
is the power supply for the metabolic pathway that’s going to produce this drug. Then we need all of the components, all the genes that will go into engineering that microbe to transform something like sugar into farnesyl pyrophosphate or FPP, a molecule that exists in every living organism, into the molecule of choice—artemisinin acid. And to do this entire transformation we needed about twelve genes, some from yeast, some from plants, and some from bacteria like E. coli.
So we start with the first gene in the metabolic pathway, the amorphadiene synthase gene. As it turns out, the gene had been cloned and the sequence was available to us, but the person who had done the work wouldn’t give us the gene because he said we were his competitors.
We actually had to start with a different source. In this case we started with a similar gene that encoded a similar product, not the one we wanted; in this case it comes from tobacco. It’s the epi-aristolochene synthase gene. It takes the same precursor, farnesyl pyrophosphate, and turns it into something similar to amorphadiene—not the product we wanted, though. When we transformed this gene into E. coli or a chassis it actually worked incredibly poorly, and that’s shown on this plot (fig. 5).
At the top of this plot is a gram-per-liter production. And we usually figure in my laboratory that any company worth its salt ought to be able to scale this up and get up to the 25 grams per liter that we’re going to need for economical production of the drug. But we’re six orders of magnitude between where we want to be and where we are with this gene. And what’s more, it’s the wrong product!

engineering biology |
287 |
Figure 5. Production of 5-epi-aristolochene by E. coli
Since we couldn’t get access to the gene and because those genes from plants express rather poorly when you transform them into an organism like E. coli, we actually decided to use a computer to recode the gene. And the beauty of this is that we can take the gene sequence from the plant, translate it into the amino acids sequence that encodes the protein, and then back-translate into a gene sequence that might look more like a microbial gene sequence.
What you do when you back-translate these is to remove codons, the three base pair sequence of DNA or RNA that encodes an amino acid, and to remove the rare codons that you would find in a plant and put in codons that the microbe might more often use. So essentially what we’re doing is taking a plant gene and making it look like a microbial gene so that the microbe won’t reject that gene when you insert it.
And what’s more, you can get about a two order of magnitude, a one hundredfold improvement in the production, when you recode these genes and make them look like microbial genes. So this got us a great boost. We don’t always get this boost when we do this recoding, but it’s really nice when you’re looking at six orders of magnitude and you can take two-order-of-magnitude jumps.
Now this pointed to the fact that the limitations were probably no longer in this gene that we had just inserted into the cell, but were probably in the primary biochemical pathways that produce the precursor to amorphadiene, farnesyl pyrophosphate or FPP. So we needed a substitute pathway rather than the native pathway. We decided to take a pathway about which a great deal is known.
This is the mevalonate pathway. And the reason that a huge amount is known about this pathway is that in you and me this is responsible for cholesterol production. Cholesterol is a multibillion-dollar problem

288 |
jay d. keasling |
Figure 6. Yeast mevalonate pathway inserted into E. coli
and a multibillion-dollar opportunity for the pharmaceutical industry, and in fact this enzyme (fig. 6) HMG-CoA is the enzyme that most of the statins we take target to lower cholesterol. And because this pathway is so well known and the genes have been cloned from so many organisms, we could take those genes and actually put them into our microbe, our host microbe E. coli. So we took those genes from yeast, Saccharomyces cerevisiae, and essentially gave E. coli a cholesterol problem.
Now, I don’t want you to think that we just need to take eight genes and insert them into E. coli and get them to function. There’s an entire set of regulatory genes that you actually have to encode with these genes that encode the enzymes. So you need promoters that turn on the gene expression, you need DNA-binding proteins that turn off the gene expression, and all of this has to be very carefully balanced. All in all you need about forty components in order to encode this metabolic pathway. It’s a huge coordination problem because the cell still has to survive while it’s producing our product of choice.
Inserting this metabolic pathway gave us about another hundredfold improvement in production (fig. 7). But along the way it also caused some problems (and that’s shown in fig. 8). So this plot shows the growth of a microbe over time. A microbe might grow up in a shake-flask (in the fashion shown right here with the black curve). That is, it will have some exponential growth period and then it will taper off its growth.

engineering biology |
289 |
Figure 7. The yeast mevalonate pathway improves yields ~90-fold.
If you add in a single copy of that metabolic pathway, that cholesterol biosynthetic pathway, the organisms grow much more slowly, and if you put many copies in, the organisms grow really poorly.
Often the choice for engineering the metabolism inside a microbe is to put in many copies of the genes of choice, and in fact what can often occur is that the microbes won’t grow. And here’s the dilemma. If we put in too few copies they won’t produce our product; if we put in too many copies, the microbe won’t grow and you won’t produce your product, so you’ve got to optimize this metabolic pathway very carefully inside the cell.
Well, to make a long story short, an intermediate in this metabolic pathway, HMG-CoA, was accumulating inside those cells when we
Figure 8. Growth of E. coli with the engineered pathway
290 |
jay d. keasling |
put large numbers of copies of this pathway into the cell. And that HMG-CoA was an inhibitor to fatty acid biosynthesis, so the cell could not in fact produce the cell wall around it, and that caused the slow growth.
Now, one solution to this problem is that you can actually add fatty acids to the growth medium, and those get incorporated into the cell wall, and the cells will recover their growth.
The problem with the solution is that the fatty acids are more expensive than the product we are trying to produce. It’s not a great way to lower the cost of an anti-malarial drug. So we actually had to go in and engineer the pathway. By debugging this metabolic pathway we got about another fiftyfold improvement in the production. And that was close enough to our production target that we could give it to our partner Amyris Biotechnologies. Through optimization of the pathways as well as the organism in large tanks, they were able to get the production well beyond the 25 grams per liter that we needed for economical production.
But what I’ve talked to you about is just this step, stopping here at the amorphadiene biosynthetic pathway. We also had to clone out the genes that encoded P450s that actually added oxygen in just the right position to the amorphadiene to get the artemisinin acid, and that required that we go into the plant and actually clone out those genes.
What I’ve shown you is that over several years we’ve increased the production of this molecule by about ten millionfold. In doing so, we can reduce the cost of this drug about tenfold over its current cost. That will mean that we can deliver a drug to poor children in Africa at about a quarter a dose, a quarter a cure.
Now it’s fine to do the science and it’s fun to think about the science and that’s the part that I enjoy the most. But if you actually want to get this out to people so that they can benefit from the drug, you’ve also got to think about the intellectual property around this. You want companies to go and use it to actually produce the drug.
So, my laboratory patented everything that we did, and those patents are held by the University of California. But in order to get this out to people there’s not going to be much profit made. So the University of California said, “For any company that will take this drug and actually produce it, we’ll give away the patents, royalty-free, you can have them exclusively. But there’s one stipulation: you can’t make money from this. If we’re not going to make money, you can’t make money.”
Amyris Biotechnologies, the company that grew out of my laboratory based on this technology, said, “Okay. We’ll license the technology and we won’t make any money for it.” Doesn’t sound like a great model from the startup, does it?
engineering biology |
291 |
I’ll tell you about how they’re going to make money from this technology. But they did all the work, free of charge. They’re not going to make any money on it. All they got was the money from the Gates Foundation to do the optimization. And then we licensed those microbes that were well tuned to produce this drug to Sanofi Aventis.
Sanofi makes artemisinin combination therapies. But they currently get the drug from the plant and that’s where the main problem is: the fluctuation in the cost and the availability. Now they’re scaling up this process and by late 2010 and early 2011 we’ll have artemisinin combination therapies on the market using this microbe that’s been engineered to produce this drug at a significantly lowered cost. And the profits they make on selling these drugs to travelers—people who have lots of money—they’re going to use to reduce the cost of the drugs for poor children in Africa.
Now what else can we do in this technology? Well, now that we’ve finished with the anti-malarial drug project, we decided to turn our attention to another serious problem, and that is global warming. Now you all know about taking petroleum and refining that into fuels for your car and the problems that that causes in terms of CO2 production in the atmosphere.
Well, one way around this is to produce renewable fuels. By renewable, I mean that in growing a plant, that plant takes up carbon dioxide. You can then take the, in this case, starch from that plant, turn it into a sugar, and into a fuel, and when that fuel is burned, you produce carbon dioxide. So in theory you could have a carbon-neutral biofuel.
Now, you’ve probably also heard about the challenges around our current approach in the U.S. in producing fuels. One is that we’re using a crop that was actually bred to be a food crop, not an energy crop. We’d like to replace that with biomass, things like tall grasses that more efficiently fix carbon dioxide, and are much more productive. Also, we can use much more of the plant.
Probably you also know about the challenges with the fuel that we’re choosing to produce. You may not, so I’m going to talk just a bit about them.
Ethanol has been a great start, but it’s what nature gave us as a fuel, and the problem with ethanol is that it’s toxic. It’s toxic to the producer microbe—in this case, yeast—it’s only produced, at most, 20% in fermentation broth, so the yeast die after they get up to about 20%. That means that we’ve got about 80% water that we have to get out of that ethanol. We do that by distilling the ethanol out of the fermentation broth, and we do that by adding a lot of heat, which is expensive and costly in terms of energy.
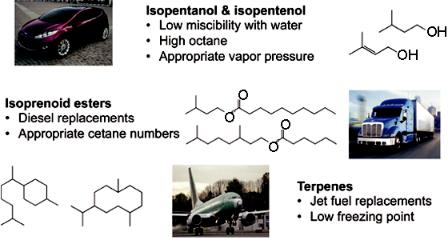
292 |
jay d. keasling |
Now, once we get that ethanol, the way we get it to the gas station is through trucks. You can’t put ethanol in pipelines because it’s corrosive to petroleum pipelines. So we have a transportation problem. And in fact one of the big challenges in getting ethanol from the Midwest to the East and West coasts where it could be used for automobiles is a limitation in train cars.
The other problem is that ethanol can be used only in limited ways in our modes of transportation. For most of you who don’t have a flex-fuel car, you can put only 10% ethanol into your gasoline; beyond that it’s corrosive to the car. It can’t be used in jet engines and it can’t be used in diesel trucks. I like to say that ethanol is for drinking, not for driving.
So if you’re going to replace ethanol, what are you going to replace it with? Well, what if we produced something that was more suited to the three trillion dollars of transportation infrastructure we have, rather than replacing that transportation infrastructure? And my argument to you now is that we’re at a point with biology where we can engineer the biology to produce the kinds of fuels that will work within our transportation infrastructure—and those fuels should be oil-based. So if we could engineer a microbe to produce an oil, that oil might be separate. Oil and water don’t mix, and what’s more, you could either put that into a refinery and refine it, just as we do with petroleum that we pump up out of the ground, or you could produce the fuel directly and put it right into your gas tank.
So these are some of the molecules that we’re considering for production (fig. 9). These molecules are alcohols that have branches in
Figure 9. Potential fuel molecules