
Reactive Intermediate Chemistry
.pdfSTRUCTURE OF CARBANIONS |
71 |
C Li bond has greater ionic character, yielding a more carbanion-like salt with higher reactivity.14 On the other hand, carbanions derived from highly acidic species such malonate esters are readily formed under mild conditions and have long been used in the generation of new carbon–carbon bonds.15–17
The modern study of carbanions as reactive intermediates and the investigation of their physical properties begins with early efforts to gauge their basicity. In their classic paper, ‘‘The Study of Extremely Weak Acids,’’ Conant and Wheland18 qualitatively explored the basicity of a range of stabilized carbanions such as polyarylmethides by allowing their sodium or potassium salts to react with a carbon acid and using color changes to indicate whether a proton transfer had occurred or not. Although crude in design relative to later studies, the results, which were later elaborated by McEwen,19 are in surprisingly good accord with modern values when the same reference points are used and the comparison is limited to moderately acidic hydrocarbons such as cyclopentadiene derivatives and polyarylmethanes. For the most basic carbanions such as those derived from simple alkanes, equilibrium methods proved to be unsuitable because of the slow kinetics of proton trans-
fer. One alternative was to explore related equilibria such as transmetalation reactions of organolithium salts with alkyl iodides.20,21 At about the same time,
kinetic methods were developed to gauge the basicity of these carbanions,
and took advantage of the Brønsted relationship between proton-transfer rate andpKa.22 Pioneering work by Shatenshtein23 and Streitwieser and co-workers24–36
using isotope-exchange rates in ammonia and cyclohexylamine, respectively, provided the first quantitative measures of basicity in carbanions derived from saturated hydrocarbons. These studies also lead to a much more detailed understanding of the proton-transfer process in solution. Cram37 brought these data together in his seminal book on carbanion chemistry and referred to it as the MSAD scale, named for the four important contributors: McEwen, Streitwieser, Applequist, and Dessy. Over time, both the equilibrium and kinetic methods evolved and yielded very accurate measures of carbanion stability in a range of solvents including dimethyl
sulfoxide (DMSO),38,39 cyclohexylamine,40–47 and ethers such as tetrahydrofuran (THF)48–51 and 1,2-dimethoxyethane (DME).52,53 These studies highlighted the
variety of ion-pairing motifs that are available to carbanion salts and provided important insights into the factors that influence condensed-phase ion-pairing behavior. Most recently, carbanions have been studied in the gas phase via mass spectrometry (MS). This work has lead to accurate data for a wide range of bare carbanions54 and allowed for an analysis of the fundamental factors that affect carbanion stability in the absence of solvation and ion-pairing effects.
3. STRUCTURE OF CARBANIONS
3.1. Geometries
For an sp3 hybridized carbanion, one expects that it will prefer a structure where the central carbon adopts a trigonal-pyramidal geometry with a lone-pair occupying
72 CARBANIONS
one of the typical tetrahedral valencies. This result is in direct analogy to a nitrogencontaining species like NH3, a compound that is isoelectronic with CH3 . This assumption has been confirmed experimentally for the methyl anion by photoelectron spectroscopy (PES) measurements that suggest a barrier to inversion and therefore a pyramidal structure.55 Although it is experimentally difficult to explore the structural details of bare carbanions, a great deal has been discovered through computational work.56 High-level work on the methyl anion indicates a pyramidal
structure with H C H angles (109.4 ) very much like those found in CH4 |
(i.e., |
||
|
57 |
|
˚ |
a tetrahedral arrangement). |
|
The C H bond lengths are stretched to 1.101 A in |
˚
CH3 (cf. 1.077 A for the methyl radical) most likely as a mechanism for dispersing the charge. The calculated barrier to inversion is 2.1 kcal/mol, which is about 4 kcal/mol smaller than that seen in NH3.58 The smaller barrier to inversion in the methyl anion can be attributed to the more diffuse nature of the carbon lone pair that gains less stabilization from introduction of s character into the orbital. In other words, the orbital contraction that occurs in going from a pure p (planar carbanion) to an sp3 hybridized lone pair (pyramidal) has a smaller energetic effect because the lone-pair electrons are more dispersed in the carbanion. The low electronegativity of carbon also is evident in the electron binding energy of CH3 , a mere 1.8 kcal/mol.55 In fact, computational work indicates that other simple alkyl anions like ethyl and isopropyl are unbound (i.e., negative electron binding energy) and would spontaneously eject an electron if they were formed in the gas phase.59 Of course, these species can be stable in the condensed phase because bonding to metals stabilizes the carbanion and effectively increases the electron binding energy.
Analogously, carbanions located at sp2 hybridized centers are expected to have bent geometries with a lone pair occupying the site of the missing proton on the previously trigonal-planar carbon. High-level computations confirm this structure and indicate a barrier to inversion of the carbanion center of 31 kcal/mol.56 Photoelectron spectroscopy on the vinyl anion gives an electron binding energy of 15.4 kcal/mol.60 The much higher electron affinity of vinyl compared to methyl can be attributed to the greater amount of s character in the orbital that forms the lone pair. This difference in hybridization also plays a role in the inversion barrier because when the carbanion adopts a linear geometry (H C CH2), the carbanion lone pair must reside in a purely p orbital and loses the advantage of sp2 hybridization. The effect of hybridization on carbanion basicity will be discussed in detail in Section 4.2.2.
The ethynyl anion provides an example of an sp hybridized carbanion. The high degree of s character in the lone-pair orbital leads to a very large electron affinity for the ethynyl group. Photoelectron spectroscopy indicates an electron binding energy of nearly 70 kcal/mol for the HC C anion.60
3.2. Stereochemistry and Racemization
For a carbanion derived from a tetrahedral carbon (i.e., sp3 hybridized) to exhibit chirality, it must either remain pyramidal or if inversion occurs, one face must

STRUCTURE OF CARBANIONS |
73 |
remain distinct from the other. The latter requirement can be attained by the presence of chiral auxiliaries apart from the carbanion center, or from a barrier to rotation in the carbanion that locks the system into a single, asymmetric conformation. The first question to be addressed is the barrier to inversion. As noted above, the inversion barrier in the bare methyl anion is exceedingly small, 2 kcal/mol, so no stereochemical integrity is expected.56 In fact, most studies have shown that simple
sp3 hybridized carbanions generally are unable to retain their asymmetry even under conditions where they react soon after being formed.61,62 Coordination of
a carbanion to a metal cation such as lithium should increase the barrier to inversion because as the carbon lone pair shifts to the opposing side of the carbon, significant electrostatic attraction is lost until the cation is able to reorient itself on the opposite side. As the carbon–metal bond becomes more covalent, this effect becomes more important and chiral mercury salts of carbanions are easily prepared and retain their asymmetry under most conditions.63 For lithium salts, a few structural motifs have been identified that lead to sp3 hybridized carbanions with appreciable stereochemical integrity. One of the earliest to be identified was the cyclopropyl carbanion. In this case, inversion is hindered by ring strain (in the inversion, the carbanion must become sp2 hybridized) and a large inversion barrier, 16.3 kcal/mol, has been predicted by high-level ab initio calculations.64 Not surprisingly, the isoelectronic, nitrogen analogue of the cyclopropyl anion, aziridine, has been shown experimentally to have an unusually large barrier to inversion (19.1 kcal/mol).65 As a result of the significant inversion barrier, the lithium salts of cyclopropyl anions can be generated in enantiomerically pure form at moderate temperatures from the action of an alkyllithium on an appropriate chiral precursor such as a halide or a stannane (direct reduction of chiral alkyl halides by lithium metal generally leads to racemization presumably due to the presence of radical intermediates66). For example, when (S) 1-methyl-2,2-diphenylcyclopropylbromide is treated with butyllithium followed by CO2 in ether or THF, there is complete retention of stereochemistry in the resulting carboxylic acid (Eq. 1).67
Br |
Li |
CO2H |
|
||
Me BuLi |
Me CO2 |
Me |
ð1Þ |
||
Ph Ph |
|
Ph Ph |
|
Ph Ph |
|
More recently, Kass and co-workers68 showed that bare cyclopropyl carbanions can be configurationally stable in the gas phase. After fluorodesilylation of the cis and trans isomers of 2-trimethylsilylcyclopropanecarboxaldehyde, they found that the isomers did not interconvert at room temperature and give different product distributions in gas-phase reactions (Scheme 3.1). High level ab initio calculations suggest an inversion barrier (15.9 kcal/mol) that is close to that of the parent cyclopropyl carbanion and consistent with configurational stability at 25 C. Although these species are diastereomers, the absence of inversion indicates that with the proper substrates (i.e., enantiomerically pure), asymmetric gas-phase cyclopropyl carbanions can be generated.
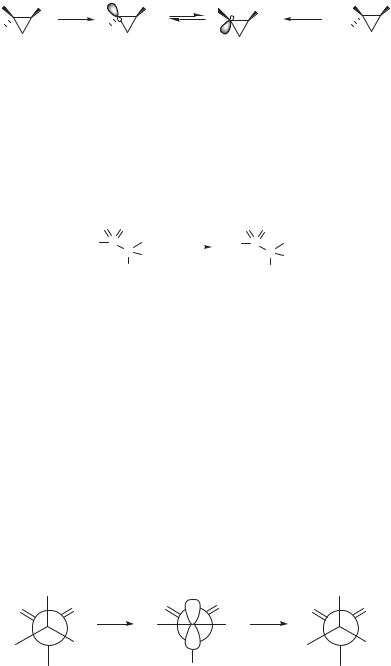
74 |
CARBANIONS |
|
|
|
|
|
Me3Si |
CHO F – |
CHO |
H |
CHO F – |
H |
CHO |
|
– |
X |
– |
|
|
|
H |
H |
|
|
|
Me3Si |
|
Scheme 3.1
Cram et al.69 and Corey et al.70 both demonstrated that salts of carbanions stabilized by an adjacent sulfone or related group can also retain their stereochemistry. For example, Cram found that a labeled, chiral 2-octyl phenyl sulfone underwent hydrogen/deuterium (H/D) exchange with the solvent >100 times faster than the optical activity was lost (Eq. 2).71 This provides strong evidence that the carbanion, once generated, maintained its stereochemical integrity long enough for solvent to protonate it and complete the H/D exchange process.
O |
|
O |
t -BuOK |
O |
|
O |
|
||
Ph |
S |
D |
Ph |
S |
H |
|
|||
*C Me |
|
|
* C Me |
|
|||||
t-BuOH |
ð2Þ |
||||||||
|
|
|
|
||||||
|
|
C6H13 |
|
|
|
|
C6H13 |
kexc /krac = 139 (25 °C)
In this case, computational evidence does not point to a large barrier to inversion,72,73 so other factors are at play. A likely explanation is that the sulfone group
prevents rotation around the C S bond. This has been confirmed by ab initio calculations that indicate a barrier of over 14 kcal/mol to C S rotation in the
MeSO2CH2 anion.72 Although the participation of d orbitals in the bonding to sulfur had been implicated in the large rotational barrier,74,75 it appears that it is
simply the result of a strong electrostatic interaction with the polar SO2 group that is maximized by aligning the carbon lone pair such that it is gauche to both sulfonyl oxygens.72 One mechanism for these carbanions to remain asymmetric is illustrated in Scheme 3.2. It is based on the following assumptions: (a) The carbanion is effectively planar (i.e., the inversion barrier, if any, allows for rapid interconversion); (b) the C S rotational barrier locks the carbanion into one conformation; and (c) the departing and returning group (deuteron and proton in this example) must exit and enter from the same face (gauche to both oxygens in this drawing). This analysis is generally supported by the available evidence.76
|
|
|
planar |
|
|
|
|
D |
|
carbanion |
|
|
H |
O |
O |
O |
O |
|
O |
O |
t -BuOK |
|
t -BuOH |
||||
|
|
|
|
|
||
|
|
R1 |
|
R2 |
|
|
R |
R2 |
|
|
|
R |
R2 |
1 |
|
|
|
|
1 |
|
|
R |
|
R |
|
|
R |
|
|
|
|
|
Scheme 3.2
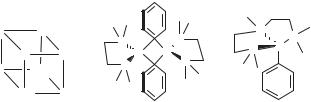
STRUCTURE OF CARBANIONS |
75 |
3.3. Magnetic Properties and Nuclear Magnetic Resonance
Nuclear magnetic resonance (NMR) has proven to be a very powerful technique for probing the structures of carbanions in the condensed phase.77–82 In particular, much work has been completed on the ion-pairing behavior of carbanions with lithium cations as well as the formation of aggregates of these lithium salts. A full discussion of this topic, particularly the methodology, is beyond the scope of this chapter, but a brief overview is appropriate.
The carbanion center is naturally electron-rich so NMR signals associated with it will appear unusually far upfield. For example, isopropyllithium gives an hydrogen NMR (1H NMR) around 0.7 ppm for the proton on the secondary carbon.77 Similarly, the 13C NMR signal for a carbanion is also upfield from tetramethylsilane (TMS) and methyllithium gives a carbon resonance in the range of 10 to 20 ppm depending on the solvent and temperature. The other unusual feature that NMR reveals about the lithium salts of carbanions is the formation of aggregates (oligomers) in solution. The aggregation of lithium salts has also been confirmed by colligative measurements,83,84 crystallography,85,86 and computational modeling.87 The high charge densities of the lithium cation and carbanion combined with the low dielectric constants of the appropriate NMR solvents (i.e., hydrocarbons and ethers) lead to a situation where electrostatic forces favor the formation of dimers, tetramers, and higher aggregates. A classic example is methyllithium, which has been shown by a number of techniques to be a tetramer in solvents like diethyl ether, 1.88,89 This arrangement allows each lithium cation to interact with three methyl anions and vice versa. The resulting, strong electrostatic stabilizations outweigh the obvious entropic disadvantage of a polymeric structure. The equilibria involving the lithium salts can be dynamic with mixtures of monomers, dimers, and higher aggregates coexisting. The position of the equilibria as well as the rate constants for interconversion between aggregates are naturally dependent on the nature of the solvent, the temperature, and any added ligands. For example, phenyllithium is mainly a dimer, 2, in a mixture of diethyl ether and tetramethylethylenediamine (Me2NCH2CH2NMe2, TMEDA), but is monomeric, 3, when dissolved in THF containing pentamethyldiethylenetriamine (Me2NCH2CH2NMeCH2CH2NMe2).77,90
Me |
Li |
|
N |
|
N |
N |
N |
|
|
|
|
|
|||
|
|
|
|
N |
Li |
||
|
Li |
Me |
Li |
Li |
|
||
|
|
|
|||||
|
N |
|
|
||||
Li |
Me |
|
N |
|
|
|
|
|
|
|
|
|
|||
|
|
|
|
|
|
||
|
Me |
Li |
|
|
|
|
|
|
1 |
|
2 |
|
|
|
3 |
The situation becomes even more complex when other lithium salts are present in the solution. For example, when a lithium halide such as LiBr is added to solutions of alkyllithiums, mixed aggregates are formed where a bromide anion takes the
76 CARBANIONS
place of a carbanion.91–94 These mixed aggregates may play important roles in synthetic chemistry because alkyllithiums are often formed by the action of lithium metal on an alkyl bromide so LiBr is a common contaminant. Kinetic studies have shown that the presence of organolithium aggregates or halide-containing mixed aggregates has a significant impact on reaction rates.95–97
4. BASICITY OF CARBANIONS–ACIDITY OF CARBON ACIDS
The most widely studied physical property of carbanions is their basicity, which of course is a direct measure of the acidity of the parent carbon acid. Carbon acidity measurements date back to the early part of the twentieth century and a myriad of techniques have been employed for the measurements. Although early measurements were only able to provide semiquantitative data, more recent ones have resulted in accurate acidity measurements across a vast range of effective acid dissociation constants, Ka values. This section will begin with a brief description of definitions and methodologies followed by representative data as well as applications of those data.
4.1. Definitions and Methodologies
Acidity has been most widely studied in aqueous solution and is easily defined in this medium by the acid dissociation equilibrium constant, Ka (Eq. 3) where S represents the solvent employed in the measurement. Given the magnitudes of Ka values, they are often expressed as pKa values for convenience (Eq. 4).
Ka |
ð3Þ |
AH þ S Ð A þ SHþ |
|
pKa ¼ logKa |
ð4Þ |
One assumption of this treatment is that the role of the solvent is limited to acting as a solvating agent for the proton and the other species in the equilibrium. In other words, the solvent cannot react with either the parent acid (AH) or its conjugate base (A ). Because carbanions are often exceptionally strong bases, the requirement that they do not react with the solvent is a major limitation. This limitation is known as the leveling effect98 and simply stated, it is not possible to use equilibrium techniques to measure the acidity of a species that is a weaker acid than the solvent because the resulting carbanion would be able to deprotonate the solvent. Very few carbon acids are more acidic than water, so measurements involving these species most often have been completed in other, less acidic solvents.
The next issue that arises from the weak acidity of carbon acids involves the degree of self-dissociation. In Eq. 3, the equilibrium constant is determined by measuring the concentration of the four species in the equation, but this requires that the carbon acid self-dissociates to an extent that a measurable quantity of the carbanion is formed. Again, because carbon acids are generally weak, this requirement often is not met and therefore another type of equilibrium measurement

BASICITY OF CARBANIONS–ACIDITY OF CARBON ACIDS |
77 |
must be employed. In this case, a measurable concentration of the carbanion is generated in solution by the addition of a very strong base, and the acidity is measured relative to another species of similar acidity (Eq. 5). The solvent no longer appears in this equilibrium expression, but it does play a role through its solvation of the other species in the equilibrium, especially the anions. If one of the species in the equilibrium measurement has a known Ka value, then the relative measurement from Eq. 5 can be converted to an absolute value (i.e., pKa).
A1H þ A2 |
|
Krel |
A1 þ A2H |
ð5Þ |
|
|
|
|
|||
|
|
A common technique for measuring the Krel values has been to employ species that produce anions with useful ultraviolet (UV) or visible (vis) absorbances and then determine the concentrations of these species spectrophotometrically. Alternatively, NMR measurements could be employed, but generally they require higher concentrations than the spectrophotometric methods. A hidden assumption in Eq. 5 is that the carbanion is fully dissociated in solution to give a free anion. Of course, most simple salts do fully dissociate in aqueous solution, but this is not necessarily true in the less polar solvents that are typical employed with carbanion salts. For example, dissociation is commonly observed for potassium salts of carbanions in DMSO because the solvent has an exceptionally large dielectric constant (e ¼ 46:7) and solvates cations very well, whereas dissociation occurs to a small extent in common solvents such as DME and THF (dielectric constants of 7.2 and 7.6, respectively). In these situations, the counterion, Mþ, plays a role in the measurements because it is the relative stability of the ion pairs that determines the position of the equilibrium constant (Eq. 6).
A |
H |
þ |
A Mþ |
|
Krel |
A Mþ |
þ |
A H |
6 |
Þ |
|
|
|
|
|||||||||
1 |
|
2 |
|
|
|
1 |
2 |
ð |
Here, the measurements lead to values that are best described as ‘‘ion-pair’’ acidities. Although it is not possible to directly relate these values to true pKa values because they do not reflect the stability of the bare carbanion, they do provide useful data, particularly for very weak acids, and are relevant to many synthetic applications of carbanions because the solvents and concentrations employed in synthetic work generally lead to ion pairing.
In the case of very weak acids, it has not been possible to find suitable conditions for establishing and monitoring equilibria such as those shown in Eqs. 5 and 6. The carbanions react too readily with the solvent or equilibrium with the reference acid (i.e., A1H) is reached too slowly for practical measurements. Here, kinetic measurements have been employed. These studies rely on the presence of a Brønsted relationship22 between the rate of deprotonation of a carbon acid and the stability
of the resulting carbanion. This correlation has proven to be viable in many instances and kinetic acidities are available in a range of solvents.23,99,100 In gen-
eral, these methods have used isotope exchange to monitor the rate of deprotonation. For example, when a mixture of a-deuterated and tritiated toluene (PhCH2D or PhCH2T) is treated with lithium cyclohexylamide (c-C6H11NHLi) in cyclohexylamine for an extended time period, the disappearance of the isotopic labels gives
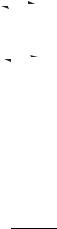
78 CARBANIONS
a measure of both the deprotonation rate as well as the primary isotope effect in the deprotonation.99,101 When applied to a set of compounds of unknown acidity, the measurements provide a qualitative ranking of their acidities. However, if the acidities of some of the species are already known, then these can be used to establish a linear Brønsted relationship, and the acidities of the unknown species can be determined by interpolation or extrapolation from the data in the Brønsted plot. One complication in kinetic measurements of acidity is the possibility of internal return. In this case, the isotopic label is returned to the acid rather than being replaced by a proton.
AD þ B |
|
|
|
k1 |
|
A DB |
ð7aÞ |
|||
|
|
|
|
|
|
|
|
|||
|
k |
1 |
|
|
||||||
|
|
|
|
|
|
|
|
|
|
|
|
|
|
|
k2 |
|
|
ð7bÞ |
|||
A DB þ HB ! A HB þ DB |
||||||||||
A HB |
|
|
|
|
|
|
|
AH þ B |
ð7cÞ |
|
|
|
|
|
|
|
|
||||
|
|
|
|
|
|
This situation is illustrated in Eq. 7 where B represents the conjugate base of the solvent, BH, used in the kinetic experiment. After the base, B , removes the deuteron from the acid donor, A, (Eq. 7a) it is still complexed to it. At this point, the deuterated base, DB, may diffuse away and be replaced by a proton-bearing analogue, HB (Eq. 7b), or it can return the deuteron to the conjugate base of the acid. Under the typical conditions, the step in Eq. 7b is irreversible (the concentration of HB is always much greater than DB) and the rate of isotope exchange can be expressed in terms of the following elementary rate constants.
k1k2 |
ð8Þ |
kobs ¼ k2 þ k 1 |
If DB quickly diffuses away (k2 is large compared to k 1), the expression simplifies to kobs ¼ k1 and is solely dependent on the rate of deuteron abstraction. In other words, the isotopic exchange rate truly reflects the rate of deuteron abstraction. If k2 is relatively small, then the expression simplifies to kobs ¼ k1k2=k 1 and the isotope exchange kinetics are also dependent on the competition between diffusion (k2) and internal return (k 1). Internal return can be identified by the absence of an isotope effect in the exchange process. This occurs because k2 is not expected to have a significant isotope effect and the primary isotope effects inherent to k1 and k 1 are expected to nearly cancel. Streitwieser’s use of dual labeled systems (deuterium and tritium) allows isotope effects to be measured in the exchange experiment (i.e., kD=kT) and internal return can be identified directly.26
Carbanion stability has also been determined in the gas phase using mass spectrometric techniques. This allows for a measure of the intrinsic stability in the absence of any solvation effects. Given the low dielectric of a vacuum, selfionization is not energetically viable. For example, dissociation of H2O in the gas phase is endoergic by 384 kcal/mol,54 corresponding to a pKa value of >250! Consequently, acidity measurements have generally relied on measuring relative stabilities (Eq. 5) and then linking these measurements to a compound whose absolute acidity has been measured independently (usually indirectly via a
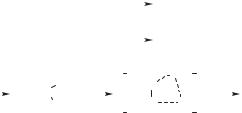
BASICITY OF CARBANIONS–ACIDITY OF CARBON ACIDS |
79 |
combination of spectroscopic and kinetic measurements, see Section 4.4). Given that self-ionization is so endothermic in a gas-phase environment, acidities under these conditions are more conveniently characterized by energy changes rather than equilibrium constants (i.e., Ka). Either the free energy ( Gacid) or enthalpy ( Hacid) of deprotonation can be used as a measure of the acidity (Eq. 9). Proton affinity (PA) is often used to describe the stability of an anion in the gas phase and is equivalent to the Hacid value for the parent acid (Eq. 10).
AH |
Gacid |
A |
þ |
Hþ |
9 |
Þ |
|
! |
|
|
ð |
||
|
or Hacid |
|
|
|
|
|
PAðA Þ ¼ HacidðAHÞ |
ð10Þ |
Measurements have been completed in the gas phase for a very wide range of organic species and for carbon acids, Gacid values have been measured that range from a low of 289 kcal/mol for a highly fluorinated bis(sulfone), (CF3CF2CF2CF2SO2)2CH2, to 409 kcal/mol for methane.54 This represents an astounding, 80 orders-of-magnitude variation in the effective equilibrium constant for deprotonation. Kinetic methods have also been developed for estimating gasphase acidities and they will be discussed in Section 4.2.1.
4.2. Structural Effects on Carbanion Basicity–Carbon Acidity
4.2.1. Carbanions Derived from sp3 Hybridized C H Bonds. There are very limited data in the gas phase or in solution on the equilibrium acidity of saturated hydrocarbons. Instead, the most comprehensive set of data comes from gasphase kinetic measurements by DePuy et al.102 Although it is not clear whether the values are quantitatively accurate across the whole series of compounds,103 the work provides a good qualitative picture of structural effects in the simplest and weakest of the carbon acids. The method relies on measuring branching ratios in the reaction of HO with a trimethylsilyl derivative of the alkane. In the process, either the unique alkane (Eq. 11a) or methane (Eq. 11b) is expelled to give a siloxide anion. Relying on the fact that the transition states for these reactions have a high degree of carbanion character (Eq. 12), it is possible to relate the branching ratio to the relative acidities of the expelled groups and develop a correlation based on species of known acidity. This approach is a variation of the kinetic acidity methods described above and is closely related to a common gas-phase methodology, the Cooks kinetic method.104
|
|
|
|
|
|
|
|
|
|
Me3SiO – + |
RH |
ð11aÞ |
|||||
|
|
Me 3Si |
|
R |
HO– |
|
|
||||||||||
|
|
|
|
|
|
|
RMe2SiO – + CH4 |
ð11bÞ |
|||||||||
|
|
|
|
|
|
|
|
|
|
||||||||
|
|
|
|
|
|
|
|
|
|
||||||||
|
HO – |
– |
OH |
|
|
H |
|
|
‡ |
|
|
|
|||||
|
|
|
|
|
|
|
|||||||||||
|
|
|
O |
|
|
|
|
|
Me3SiO– + RH |
ð12Þ |
|||||||
Me3SiR |
|
Me3Si |
|
|
|
|
Me3Si |
R – |
|
|
|
|
|||||
|
R |
|
|
|
|
|
|||||||||||
|
|
|
|
|
|
|
|
|
|
|
|
|
|
|
|
|
|

80 CARBANIONS
TABLE 3.1. Gas-Phase Hacid Values of Simple Alkanesa
Alkaneb |
Hacid (exp)c |
Hacid (comp)d |
|
|
|
CH4 |
417 |
418 |
CH3CH3 |
420 |
421 |
CH3CH2CH3 |
416 |
416 |
CH3CH(CH3)2 |
413 |
|
CH3C(CH3)3 |
409 |
413e |
MeCH2Me |
419 |
418 |
Me3CH |
413 |
412 |
c-C3H6 |
412 |
416e |
c-C4H8 |
417 |
415e |
c-C5H10 |
416 |
414e |
a In kilocalories per mole (kcal/mol). b Site of deprotonation is in bold.
c Values from gas-phase kinetic experiments (see Ref. 102). d Values from G2 calculations (see Ref. 105).
e Values from MP2/6-31 þ G** calculations (see Ref. 106).
Data for a representative set of simple alkanes are given in Table 3.1 along with values calculated at the G2105 or MP2106 levels of theory. There is a good correspondence in these systems between the kinetic and computed acidities, but it should be noted that DFT calculations106 at the B3LYP/6-311þþG(2d,p) level on these systems give Hacid values that tend to be 3–5 kcal/mol smaller than the MP2, G2, or experimental values. Starting with methane, the effect of adding methyl groups to the a-carbon can be evaluated with the acidities of ethane, propane (2 position), and isobutane (3 position). One might expect two effects from the added methyl groups. First, they are polarizable and therefore could stabilize the
resulting carbanions by effectively dispersing the charge. Second, methyl groups are viewed as electron donors in Hammett-type correlations107,108 (they have nega-
tive s-values) so they should destabilize an adjacent carbanion. It can be seen in Table 3.1 that adding the first methyl group (i.e., ethane) reduces the acidity (i.e., increases Hacid). However, it is not a simple trend and the addition of more methyl groups to the carbanion center (i.e., the isopropyl and tert-butyl carbanions) eventually leads to an increase in acidity. DePuy et al.102 has argued that the trend can be explained by competing effects where destabilization from the electron-donating characteristics of the methyl group quickly is saturated and then overwhelmed by stabilization gained from the polarizability of the additional methyl groups. A simpler trend is observed in the addition of methyl groups at the b-carbon. Here, polarizability is the main factor and the acidity increases with each added methyl group. Across the series from ethane to neopentane, there is a smooth drop in the Hacid values from 420 to 409 kcal/mol with each methyl group causing about a 3-kcal/ mol increase in acidity. Data in Table 3.1 also indicate that ring strain can increase acidity. Specifically, cyclopropane is much more acidic than other monocyclic hydrocarbons or the secondary position of a simple alkane (i.e., propane). It is