
- •Preface
- •Contents
- •1 Introduction
- •Layered Materials and Their Electronic Structure
- •General Phase Diagram of Cuprates and Main Questions
- •Superconducting State: Symmetry of the Order Parameter
- •Triplet Pairing in Strontium Ruthenate (Sr2RuO4): Main Facts and Main Questions
- •From the Crystal Structure to Electronic Properties
- •Spin Fluctuation Mechanism for Superconductivity
- •References
- •Generalized Eliashberg Equations for Cuprates and Strontium Ruthenate
- •Theory for Underdoped Cuprates
- •Extensions for the Inclusion of a d-Wave Pseudogap
- •Derivation of Important Formulae and Quantities
- •Elementary Excitations
- •Raman Scattering Intensity Including Vertex Corrections
- •Optical Conductivity
- •Comparison with Similar Approaches for Cuprates
- •The Spin Bag Mechanism
- •Other Scenarios for Cuprates: Doping a Mott Insulator
- •Local vs. Nonlocal Correlations
- •The Large-U Limit
- •Projected Trial Wave Functions and the RVB Picture
- •Current Research and Discussion
- •References
- •The Spectral Density Observed by ARPES: Explanation of the Kink Feature
- •Raman Response and its Relation to the Anisotropy and Temperature Dependence of the Scattering Rate
- •A Reinvestigation of Inelastic Neutron Scattering
- •Collective Modes in Electronic Raman Scattering?
- •Elementary Excitations and the Phase Diagram
- •Optical Conductivity and Electronic Raman Response
- •Brief Summary of the Consequences of the Pseudogap
- •References
- •4 Results for Sr2RuO4
- •Elementary Spin Excitations in the Normal State of Sr2RuO4
- •The Role of Hybridization
- •Comparison with Experiment
- •Symmetry Analysis of the Superconducting Order Parameter
- •Triplet Pairing Arising from Spin Excitations
- •Summary, Comparison with Cuprates, and Outlook
- •References
- •5 Summary, Conclusions, and Critical remarks
- •References
- •References
- •Index
12 1 Introduction
Let us briefly summarize the main questions for the normal state of cuprates:
–How can we understand the phase diagrams of both for hole– and electron–doped cuprates, in particular the doping dependence of the char-
acteristic temperatures T (x), Tc (x), and Tc(x)? What is the origin of the pseudogap? Do T and Tc exist for electron-doped cuprates?
–What is the origin of the asymmetry of the cuprate phase diagram with respect to hole and electron doping? Why does superconductivity occur only within a small doping region, and why does it have a smaller Tc in the case of electron-doped cuprates?
–How can we describe and understand the elementary excitations in cuprates, for example the kink feature in hole–doped cuprates and its absence in electron–doped cuprates?
Finally Fig. 1.4 illustrates that (mainly AF) spin fluctuations are present in the normal state of hole–doped cuprates; these can be measured by INS experiments, for example [43]. Spin fluctuations have also been measured in electron-doped cuprates [44]; however, they are weaker than in hole–doped cuprates. As already mentioned, their occurrence is related to the quasi– 2D character of the spin correlations within a CuO2 plane, which are more robust against doping than is the 3D N´eel state. The origin of these excitations in hole–doped cuprates is the copper spins, which are surrounded by itinerant holes which have destroyed the 3D long-range order. Consequently, the underlying idea for the Cooper-pairing mechanism in high-Tc cuprates is the exchange of these spin fluctuations between (dressed) holes or electrons in a generalized Eliashberg-like theory. This will be discussed in detail in Chap. 2. These ideas are similar to the exchange of “paramagnons” in the case of triplet pairing in 3He, where the system is close to a ferromagnetic instability [45, 46]. Later in this book we shall use a similar approach to describe the interesting properties of the novel triplet superconductor Sr2RuO4.
1.2.2 Superconducting State: Symmetry of the Order Parameter
In Fig. 1.4, we also show the Tc(x) curve, which has a characteristic shape Tc(x) = 1 − (x − 0.16)2, as pointed out in [17, 47]. Below Tc, superconductivity occurs and it is believed that the order parameter has dx2−y2 –wave symmetry, i.e. ∆k = ∆0 [cos kx −cos ky ]/2. The evidence for d–wave pairing in hole–doped cuprates comes from several sources, in particular phase–sensitive measurements, NMR studies, penetration depth measurements, ARPES, and polarization-dependent Raman scattering experiments:
1.Phase sensitive experiments by Wollmann et al. [48] and Kirtley and Tsuei [49, 50, 51], measuring the phase coherence of YBCO–Pb dc SQUIDs, have reported a dx2−y2 -wave order parameter.

1.2 General Phase Diagram of Cuprates and Main Questions |
13 |
2.Bourges, Regnault, Keimer, and others have demonstrated that INS experiments reveal a feedback e ect of superconductivity on the neutron scattering intensity. In particular, a strong rearrangement of the spectral
weight, a so–called resonance peak, is observed below Tc (see Fig. 1.7) [52, 53, 54, 55, 56].1 On general grounds, one expect this peak at a resonance frequency ωres ≈ 2∆ where ∆ is the (average) superconducting gap. INS experiments show intensity below this threshold frequency (i.e. 0 < ω < ωres) also supporting a d–wave gap in the superconducting state.
3.NMR measurements probe the local magnetic field around an atom and
allow the determination of the Cu relaxation rate. Below Tc this relaxation rate varies as T 3, in agreement with several predictions for a dx2−y2 – wave order parameter.
4.It follows from simple statistical arguments that the penetration depth λ of an external magnetic field varies exponentially with T at small temperatures. However, when nodes are present in the superconducting or-
der parameter, and thus Cooper pairs can be broken very easily along the corresponding directions in the Brillouin zone (BZ), λ should vary linearly with temperature (or λ T 2 in the dirty limit). Bonn, Hardy, and coworkers have reported such behavior in YBCO [57, 58].
5.Shen et al. and Campuzano et al. have reported strong anisotropy of the superconducting gap using ARPES techniques [59]. Their interpretation is consistent with a dx2−y2 –wave order parameter.
6.Polarization-dependent Raman scattering below Tc measures a pairbreaking peak and thus (via the Tsuneto function) [60, 61]) the anisotropy of ∆k . So far, the interpretations of several groups are compatible with an order parameter that has nodes along the diagonal of the BZ [62, 63, 64, 65]. Further analytical results for small transferred energies, i.e. power laws for the observed intensity, support this interpretation.
Obviously, only method 1 reveals clearly a sign change in the superconducting order parameter; the other techniques can determine only the existence of nodes. Strictly speaking, some of the results of these experiments would also be consistent with an extended s–wave gap, i.e. ∆k = ∆0 [cos kx + cos ky ]/2. In addition, another phase–sensitive measurement along the c direction by Li et al., which was suggested by R. Klemm, seems to be inconsistent with a dx2−y2 –wave gap [66, 67]. Very recently, this experiment has been repeated and improved and a d–wave gap has been observed [68]. However, there is still controversy over the interpretation [69]. Furthermore, we show throughout this book that a d–wave order parameter occurs naturally if singlet pairing is mediated by AF spin fluctuations. This is related to general arguments about a repulsive pairing interaction and will be discussed in Sect. 1.4.3.
1A closer inspection for the normal-state data of underdoped YBa2Cu3O6+x [55] shows that this peak is qualitatively di erent from the resonance peak [56].
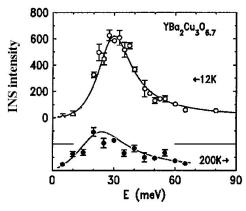
14 1 Introduction
Fig. 1.7. Neutron scattering intensity versus transferred energy for underdoped YBCO in the normal state (T = 200 K) and superconducting state (T = 12 K). In the normal state, an Ornstein–Zernicke behavior is observed. Below Tc , a strong rearrangement of spectral weight takes place and a resonance peak develops.
New phase–sensitive measurements by Kirtley and Tsuei show strong evidence that the superconducting order parameter of electron–doped cuprates also has dx2−y2 –wave symmetry [51, 70]. This is further supported by measurements of the in–plane penetration depth λ, and ARPES experiments [71, 72]. This is interesting because it was believed for more than one decade that NCCO and other electron–doped superconductors were s–wave superconductors. In particular, early experiments by Anlage et al. reported that λ follows an exponential behavior at low temperatures [73], Raman scattering experiments saw no variation of the scattered intensity as a function of the applied polarization [74], and no zero–bias peak has been observed [75]. However, after the recent experiments by Kirtley and Tsuei mentioned above, it seems clear now that d–wave pairing is present in electron–doped cuprates. We consider this as an important step towards a unified phase diagram of hole– and electron–doped cuprates.
We can briefly summarize the questions as follows:
–Why, theoretically, should a dx2−y2 –wave gap appear in the case of singlet pairing due to (repulsive) spin excitations for both hole– and electron– doped cuprates? Can we exclude a dxy symmetry, for example? How is this related to the underlying band structure or the character of the quasiparticles (copper d states versus oxygen p states)?
–Why did earlier experiments on electron–doped cuprates report an s–wave symmetry of the superconducting order parameter?
–In general, if the order parameter has dx2−y2 –wave symmetry, why are deviations from the simple basis function [cos kx − cos ky ] still possible?