
- •Radio Engineering for Wireless Communication and Sensor Applications
- •Contents
- •Preface
- •Acknowledgments
- •1 Introduction to Radio Waves and Radio Engineering
- •1.1 Radio Waves as a Part of the Electromagnetic Spectrum
- •1.2 What Is Radio Engineering?
- •1.3 Allocation of Radio Frequencies
- •1.4 History of Radio Engineering from Maxwell to the Present
- •2.2 Fields in Media
- •2.3 Boundary Conditions
- •2.4 Helmholtz Equation and Its Plane Wave Solution
- •2.5 Polarization of a Plane Wave
- •2.6 Reflection and Transmission at a Dielectric Interface
- •2.7 Energy and Power
- •3 Transmission Lines and Waveguides
- •3.1 Basic Equations for Transmission Lines and Waveguides
- •3.2 Transverse Electromagnetic Wave Modes
- •3.3 Transverse Electric and Transverse Magnetic Wave Modes
- •3.4 Rectangular Waveguide
- •3.4.1 TE Wave Modes in Rectangular Waveguide
- •3.4.2 TM Wave Modes in Rectangular Waveguide
- •3.5 Circular Waveguide
- •3.6 Optical Fiber
- •3.7 Coaxial Line
- •3.8 Microstrip Line
- •3.9 Wave and Signal Velocities
- •3.10 Transmission Line Model
- •4 Impedance Matching
- •4.1 Reflection from a Mismatched Load
- •4.2 Smith Chart
- •4.3 Matching Methods
- •4.3.1 Matching with Lumped Reactive Elements
- •4.3.4 Resistive Matching
- •5 Microwave Circuit Theory
- •5.1 Impedance and Admittance Matrices
- •5.2 Scattering Matrices
- •5.3 Signal Flow Graph, Transfer Function, and Gain
- •6.1 Power Dividers and Directional Couplers
- •6.1.1 Power Dividers
- •6.1.2 Coupling and Directivity of a Directional Coupler
- •6.1.3 Scattering Matrix of a Directional Coupler
- •6.1.4 Waveguide Directional Couplers
- •6.1.5 Microstrip Directional Couplers
- •6.2 Ferrite Devices
- •6.2.1 Properties of Ferrite Materials
- •6.2.2 Faraday Rotation
- •6.2.3 Isolators
- •6.2.4 Circulators
- •6.3 Other Passive Components and Devices
- •6.3.1 Terminations
- •6.3.2 Attenuators
- •6.3.3 Phase Shifters
- •6.3.4 Connectors and Adapters
- •7 Resonators and Filters
- •7.1 Resonators
- •7.1.1 Resonance Phenomenon
- •7.1.2 Quality Factor
- •7.1.3 Coupled Resonator
- •7.1.4 Transmission Line Section as a Resonator
- •7.1.5 Cavity Resonators
- •7.1.6 Dielectric Resonators
- •7.2 Filters
- •7.2.1 Insertion Loss Method
- •7.2.2 Design of Microwave Filters
- •7.2.3 Practical Microwave Filters
- •8 Circuits Based on Semiconductor Devices
- •8.1 From Electron Tubes to Semiconductor Devices
- •8.2 Important Semiconductor Devices
- •8.2.1 Diodes
- •8.2.2 Transistors
- •8.3 Oscillators
- •8.4 Amplifiers
- •8.4.2 Effect of Nonlinearities and Design of Power Amplifiers
- •8.4.3 Reflection Amplifiers
- •8.5.1 Mixers
- •8.5.2 Frequency Multipliers
- •8.6 Detectors
- •8.7 Monolithic Microwave Circuits
- •9 Antennas
- •9.1 Fundamental Concepts of Antennas
- •9.2 Calculation of Radiation from Antennas
- •9.3 Radiating Current Element
- •9.4 Dipole and Monopole Antennas
- •9.5 Other Wire Antennas
- •9.6 Radiation from Apertures
- •9.7 Horn Antennas
- •9.8 Reflector Antennas
- •9.9 Other Antennas
- •9.10 Antenna Arrays
- •9.11 Matching of Antennas
- •9.12 Link Between Two Antennas
- •10 Propagation of Radio Waves
- •10.1 Environment and Propagation Mechanisms
- •10.2 Tropospheric Attenuation
- •10.4 LOS Path
- •10.5 Reflection from Ground
- •10.6 Multipath Propagation in Cellular Mobile Radio Systems
- •10.7 Propagation Aided by Scattering: Scatter Link
- •10.8 Propagation via Ionosphere
- •11 Radio System
- •11.1 Transmitters and Receivers
- •11.2 Noise
- •11.2.1 Receiver Noise
- •11.2.2 Antenna Noise Temperature
- •11.3 Modulation and Demodulation of Signals
- •11.3.1 Analog Modulation
- •11.3.2 Digital Modulation
- •11.4 Radio Link Budget
- •12 Applications
- •12.1 Broadcasting
- •12.1.1 Broadcasting in Finland
- •12.1.2 Broadcasting Satellites
- •12.2 Radio Link Systems
- •12.2.1 Terrestrial Radio Links
- •12.2.2 Satellite Radio Links
- •12.3 Wireless Local Area Networks
- •12.4 Mobile Communication
- •12.5 Radionavigation
- •12.5.1 Hyperbolic Radionavigation Systems
- •12.5.2 Satellite Navigation Systems
- •12.5.3 Navigation Systems in Aviation
- •12.6 Radar
- •12.6.1 Pulse Radar
- •12.6.2 Doppler Radar
- •12.6.4 Surveillance and Tracking Radars
- •12.7 Remote Sensing
- •12.7.1 Radiometry
- •12.7.2 Total Power Radiometer and Dicke Radiometer
- •12.8 Radio Astronomy
- •12.8.1 Radio Telescopes and Receivers
- •12.8.2 Antenna Temperature of Radio Sources
- •12.8.3 Radio Sources in the Sky
- •12.9 Sensors for Industrial Applications
- •12.9.1 Transmission Sensors
- •12.9.2 Resonators
- •12.9.3 Reflection Sensors
- •12.9.4 Radar Sensors
- •12.9.5 Radiometer Sensors
- •12.9.6 Imaging Sensors
- •12.10 Power Applications
- •12.11 Medical Applications
- •12.11.1 Thermography
- •12.11.2 Diathermy
- •12.11.3 Hyperthermia
- •12.12 Electronic Warfare
- •List of Acronyms
- •About the Authors
- •Index

128 Radio Engineering for Wireless Communication and Sensor Applications
6.2 Ferrite Devices
Ferrites are ceramic materials that possess a high resistivity and that behave nonreciprocally when embedded in a magnetic field. Ferrite devices such as isolators, circulators, attenuators, phase shifters, modulators, and switches are based on these properties [3].
6.2.1 Properties of Ferrite Materials
Ferrites are oxides of ferromagnetic materials, such as iron, to which another oxide has been added as an impurity. According to their molecular structure, ferrites are divided into garnets (3M2O3 ? 5Fe2O3 ), spinels (MO ? Fe2O3 ), and hexaferrites. In garnets M is a lantanid like yttrium, gadolinium, or samarium. In spinels M is mangan, manganese, iron, zinc, nickel, or cadmium. The impurities increase the resistivity of the ferrite to as much as 1014 times higher than the resistivity of metals. Typically, the relative permittivity er is 10 to 20, whereas the relative permeability mr may be up to VHF band 1,000 or even more.
In ferromagnetic materials, strong interactions between atomic magnetic moments force them to line up parallel to each other. Ferromagnetic materials are able to retain magnetization when the magnetizing field is removed.
Atoms behave as magnetic dipoles because of the spin of their electrons. The orbital movement of the electrons about the nucleus also causes a small magnetic moment, but its effect is less important for the magnetic properties of materials. We can imagine that an electron is rotating about its axis
producing a magnetic dipole moment |
|
|
|||
| m | = |
eh |
= 9.27 |
× 10−24 Am2 |
(6.23) |
|
4p m e |
|||||
|
|
|
|
where e is the magnitude of the electron charge, h is Planck’s constant, and m e is the mass of an electron. The spin angular momentum of an electron is
| P | = |
h |
(6.24) |
|
4p |
|||
|
|
The vectors m and P point to opposite directions. The ratio of their magnitudes, the gyromagnetic ratio, is
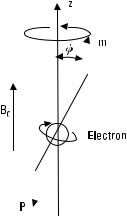
Passive Transmission Line and Waveguide Devices |
129 |
||||
g = | |
m |
| = |
e |
= 17.6 MHz/gauss |
(6.25) |
|
|
||||
|
P |
m e |
|
A static magnetic field having a flux density B0 = B0 uz exerts on an electron a torque of (m = −g P):
T = m × B0 = −g P × B0 |
(6.26) |
Because of this torque, the electrons and their magnetic dipoles precess as gyroscopes. The angle of precession, f, is shown in Figure 6.14. The rate of change of the angular momentum equals the torque, or d P /dt = T. Thus the equation of motion for a magnetic dipole is
d m |
= −gm × B0 |
(6.27) |
||
dt |
|
|||
|
|
From this we can solve the angular frequency of precession (called the Larmor frequency):
v0 = g B0 |
(6.28) |
Let us assume that both a static magnetic field with a flux density of B0 and a field of a high-frequency wave propagating into the z direction interact with an electron. The wave is circularly polarized in the xy -plane
Figure 6.14 An electron precessing in magnetic field.

130 Radio Engineering for Wireless Communication and Sensor Applications
and has a flux density of B1 (<< B0 ). For a left-handed wave, B1 = B1− = B1 (ux + j uy ). Now the total magnetic field is tilted by an angle of u = arctan (B1 /B0 ) with respect to the z -axis and precesses with the angular frequency of the wave, v . The torque due to the field produces precession of electrons in the counterclockwise direction in synchronism with the propagating wave, and therefore f < u. From the equation of motion we solve the component of the magnetic dipole moment m − that rotates in synchronism with the left-handed circularly polarized wave
m |
− |
= |
g m 0 B1 |
(6.29) |
|
v0 + v |
|||
|
|
|
|
where m 0 = m cos f . Correspondingly, for a right-handed wave B1 = B1+ = B1 (ux − j uy ), both fields produce precession in the clockwise direction, and therefore f > u, and
m |
+ |
= |
g m 0 B1 |
(6.30) |
|
v0 − v |
|||
|
|
|
|
In ferromagnetic materials, the magnetic dipole moments are aligned parallel in regions called magnetic domains, even when no external field is present. When an external field is applied, the domains tend to orient parallel to the field. The magnetization of the material, or the magnetic dipole moment per unit volume, is M = N m, where N is the effective number of electrons per unit volume. When the external field increases, nearly all magnetic moments are aligned parallel to the field and a saturation magnetization MS is finally reached. Then the whole ferrite body behaves like a large magnetic dipole. The magnetic flux density in a saturated ferrite is
B = m0 (H0 + MS ) |
(6.31) |
As for a single electron, an equation of motion can be derived for magnetization. Equations analogous to (6.29) and (6.30) are obtained for circularly polarized waves by replacing m 0 with Nm 0 . From these it follows that the effective permeabilities for right-handed and left-handed circularly polarized waves are
|
|
|
|
S |
|
v0 |
− v D |
|
|
+ |
+ |
|
|
|
gm |
0 M S |
|
m |
|
= m 0 m r |
= m 0 |
|
1 + |
|
|
(6.32) |

Passive Transmission Line and Waveguide Devices |
131 |
|
|
|
|
S |
|
v0 |
+ v D |
|
|
− |
− |
|
|
|
gm |
0 M S |
|
m |
|
= m 0 m r |
= m 0 |
|
1 + |
|
|
(6.33) |
when B1 << B0 (small-signal conditions) and M = M S . The matrix presentation is
B + |
4 |
|
3 |
m+r |
0 |
0 |
H + |
4 |
|
3Bz |
= m 0 |
0 |
0 |
1 |
43Hz |
|
|||
B − |
|
|
0 |
m−r |
0 |
H − |
|
(6.34) |
Thus the phase constant of a right-handed wave, b + = v√em+, and that of a left-handed wave, b − = v√em −, are different.
6.2.2 Faraday Rotation
Let us consider a situation in which a plane wave propagates in ferrite in the z direction. A uniform, static magnetic field pointing to the z direction is applied over the ferrite. The wave is linearly polarized and the electric field is directed along the x -axis at z = 0. A linearly polarized wave can be divided into two orthogonal circularly polarized waves, as
E = ux E0 = (ux + |
|
E0 |
+ (ux − j uy ) |
E0 |
|
|
||||||||||
j uy ) |
|
|
|
|
|
|
(6.35) |
|||||||||
|
2 |
|
2 |
|
|
|||||||||||
|
|
|
|
|
|
|
|
|
|
|
|
|
|
|
||
The phase constants of these two components are b − and b +. At |
||||||||||||||||
z = l the electric field is |
|
|
|
|
|
|
|
|
|
|
|
|
|
|
|
|
E = (ux + j uy ) |
E0 |
e |
−jb −l |
+ |
(ux − j uy ) |
E0 |
e |
−jb |
+l |
(6.36) |
||||||
2 |
|
|
2 |
|
|
|
|
|||||||||
|
|
|
|
|
|
|
|
|
|
|
|
|
|
|
This can be written as
E = E0 e −j ( b − + b + )l /2 {ux cos [( b + − b − )l /2] − uy sin [( b + − b − )l /2]} (6.37)
The phase shift of the resultant wave is ( b − + b + ) l /2 and the tilt angle with respect to the x -axis is
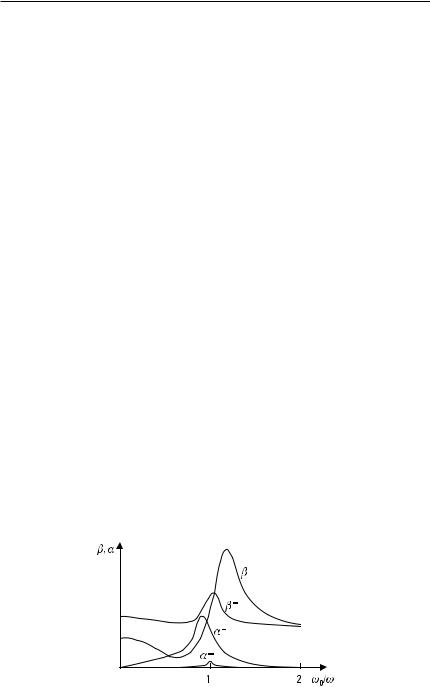
132 Radio Engineering for Wireless Communication and Sensor Applications
u = arctan (Ey /Ex ) = −( b |
+ |
− b |
− |
) |
l |
|
(6.38) |
|
|
2 |
|||||
|
|
|
|
|
|
Hence the tilt angle of the polarization vector changes as the wave propagates in a ferrite. This phenomenon is called the Faraday rotation. A typical change is 100° per centimeter at 10 GHz.
If the direction of propagation is reversed, the tilt angle rotates in the same direction with respect to the coordinate system. Therefore, as the wave propagates back from z = l to z = 0, the tilt angle does not return back from u to 0° but its value becomes 2u. Consequently, the Faraday rotation is a nonreciprocal phenomenon.
Figure 6.15 shows how the phase and attenuation constants typically
behave in a ferrite. When v 0 > v, b + > b −, and when v0 < v , b + < b −. Thus, the direction of the Faraday rotation depends on whether the signal
frequency is smaller or larger than the resonance frequency. Close to the resonance, the attenuation constant a + is large. Well below the resonance frequency, the attenuation constant is small, but then the difference between b + and b − is small and the tilt angle rotates slowly.
Example 6.2
A linearly polarized wave at a frequency of f = 3 GHz propagates in a ferrite into the direction of a static magnetic field with a flux density B0 = 0.14 Wb/m2. The ferrite has a saturation magnetization of m 0 M S = 0.2 Wb/m2 and a relative permittivity of er = 10. Find the length of such a ferrite body that rotates the tilt angle by 90° as a wave passes through it.
Solution
Using (6.25) and (6.28) we find the resonance frequency v 0 = gB0 = 24.64 × 109 1/s. Note that 1 Wb/m2 = 1 T = 104 gauss. The angular frequency
Figure 6.15 Phase and attenuation constants for propagation in ferrite.