
The Art of Genes How Organisms Make Themselves
.pdf
elaborated, eventually leading to the complex internal and external anatomy of the animal.
Fig. 14.4 Cross-section of schematic patchwork composed of overlapping patterns of hidden colour along three axes.
Telling in from out
In describing gastrulation, I skipped over a vital step. Although the dorsal-ventral pattern can distinguish those cells (flesh-red) that will form mesoderm, it does not account for why they move in rather than in any other direction. Why, for example, don't the flesh-red cells move outwards? There has to be some asymmetry that leads the cells to move specifically in an inward direction.
We can address this problem by looking in more detail at how the early cell movements occur. One of the first signs of inward movement is that the flesh-red cells become wedge-shaped, by a contraction of their outer surface, as shown in Fig. 14.5. By changing shape in this way, the cells naturally form an inward arch, starting the process of gastrulation. Now the direction of this movement dearly depends on which surface of the cells contracts: if the outer surface contracts, as illustrated in the figure, the cells arch inwards; but if the inner surface were to contract, the opposite would happen, and the cells would arch outwards. Why, then, is it only the outer surface that normally contracts? It turns out that the contraction is caused by a change in the shape of a special group of protein fibres, called contractile proteins. These proteins come to lie near to the outer surface of the cells, so it is this region that preferentially contracts (Fig. 14.5). We have therefore traced the direction of movement to an asymmetry in the distribution of contractile proteins.
Fig. 14.5
Inward movement of ventral cells caused by localised contraction.
The next logical step would be to ask how the contractile proteins come to be mainly in the outer regions of these cells. That is, how come the surface cells are themselves polarised along the inside-outside axis? The detailed answer to this is not known, but it is thought to trace back to earlier events that occur while the embryo is still a single cell. Recall that following fertilisation, the nucleus of a fruit fly egg undergoes several rounds of division to give many nuclei immersed in a common cytoplasm (Chapter 9). At this stage there is only one membrane around the cytoplasm so the embryo comprises a single cell. The nuclei then migrate to the outer regions of the cell, where they eventually become enclosed by membrane to form separate cells. This means that even before these individual cells are formed, the embryo has a defined structure from outside to inside: a membrane on the outside, cytoplasm containing nuclei further in, and then a central core. It is likely that some aspect of this earlier asymmetry between inner and outer regions of the embryo, when it is still a single cell, eventually leads to the preferential location of contractile proteins near the outer surface of the individual cells that form later on.
I have gone through this chain of events in some detail to show how the inside-outside axis can be traced back to properties of the egg cell. Basic differences in the egg, from inside to outside, lead to distinctions between inner and outer regions of the early embryo. These can then be further elaborated, in combination with the other asymmetries, such as the dorsal-ventral pattern of flesh-red, leading to the inside-outside pattern of hidden colours that distinguishes the germ layers.
I have concentrated on fly development, but many of the same considerations apply to other animals. In vertebrates, for example, an inside-outside axis is established very early on, when the embryo is a dump of a few cells (unlike the fly, the fertilised egg divides directly to give a clump of cells, without first forming a large cell with multiple nuclei inside). At this stage, particular types of protein molecules may become preferentially distributed towards the inner or outer regions of the clump. Exactly how this happens is not known but it is believed to stem from the fact that the outer surface of the clump is exposed to the surroundings, whereas the inner areas are not. Some aspect of this basic asymmetry leads to an altered distribution of molecules from inside to outside the dump. This is then built upon and elaborated, although precisely how the three germ layers are eventually established is still not dear.
There is a general lesson to be learnt from these examples. Because the early embryo is a coherent structure, a single cell or a clump of cells, there is an automatic distinction between inside and outside, between the embryo and its surroundings. This basic asymmetry can lead to particular molecules being distributed unevenly from inside to outside, eventually leading to the more elaborate inside-outside axis of the adult. Unlike the other axes of asymmetry I have mentioned, the origin of the inside-outside axis is so basic that it can almost seem trivial. We can imagine an embryo starting off without head-tail or dorsal-ventral distinctions but if it is at all coherent, it already has to have differences from inside to outside. These may be as simple as the difference between a membrane on the outside versus the cytoplasm and nucleus within, or an outer surface of a dump of cells as distinct from its interior. The particular aspect of asymmetry that is elaborated may vary from case to case, but the potential almost has to be there to begin with. In this sense, the inside-outside axis is the most fundamental of them all.
The same point can be vividly illustrated with plants. Unlike the cells of animals, plant cells are surrounded by rigid cell walls that cannot move easily relative to each other. When a fertilised plant egg cell divides, a new cell wall is laid down within it, partitioning the cell in two (Fig. 14.6). Each of these cells then divides, leading to the introduction of further partitioning walls. With each

further division, new internal partitions continue to be added in this way. But throughout this process, the original wall of the egg cell continues to surround the embryo. Although new internal walls are added, the outer foundation remains. Of course, the outer wall has to grow and expand to accommodate the enlarging embryo, but it maintains its original external location. This means that the outer surface of a mature plant traces back to the wall of the egg cell it came from (except for losses like the shedding of bark from trees and the loss of surface tissue from roots). So in this case, a basic asymmetry between outside and inside for the egg— the cell wall as distinct from its contents— becomes embodied in a corresponding asymmetry for the mature organism.
Fig. 14.6 Early development of a plant embryo. The outer cell wall is shown thicker for clarity.
Of course, mature plants are not just a bag of nondescript cells surrounded by an outer wall. Like animals, they have a complex pattern of internal tissues. Although many of the details of how this pattern develops are not yet known, it most likely reflects the elaboration of hidden colours along the inside-outside axis that traces back to a very early stage, when the embryo comprises one or a few cells. Unlike in animals, however, the elaboration process does not involve cell movement, because plant cells are held in relatively fixed positions by their cell walls. Rather, it relies on cells signalling and responding to each other.
Handedness
So far I have described how patterns can be elaborated along three different axes to give a complex patchwork that retains a plane of mirror symmetry. However, there are some aspects of plant and animal development that display a further degree of asymmetry, leaving no mirror plane at all. I now want to look at how this might come about.
Fig. 14.7
Self-portrait with Bandaged Ear (1889
),Vincent van Gogh. Cortauld Institute
, London Figure

14.7 shows a self-portrait by Vincent van Gogh with a bandaged ear. It was painted within a short time of his having had a mental breakdown, during which he cut off part of his ear. According to The Oxford Companion to Art, the picture shows that van Gogh must have mutilated his right ear, because it is clearly this one that is bandaged in the picture. A moment's ref lection, though, tells you that it actually must have been his left ear that was cut, because the picture is a self-portrait, carried out with him looking in a mirror and therefore inverting left and right. (You can also see this from the fact that the buttonhole is on the right of his jacket in the painting— the reverse of the conventional arrangement in which the buttonhole for male garments is on the left.)
Van Gogh's self-portrait makes the point that anything with asymmetry from left to right is distinct from its mirror image. But it turns out that for us to be able to distinguish an object's left and right, it also has to have asymmetries along two other axes. In the case of van Gogh's picture, for example, these are the asymmetries from top to bottom and front to back. We have no problem are applied. If we decide that the original van Gogh portrait should be called the R-form, then its mirror image would be the L-form. Such objects have no internal planes of reflection symmetry: it is not possible to find a plane running through the object that divides it into two halves that are mirror images of each other. Compare this with the situation of bilateral symmetry, shown on the right of Fig. 14.8. Here there are also three axes, but one of them, the horizontal axis in this case, acts symmetrically with respect to the other two. The object could therefore be superimposed on its mirror image, and it retains a single plane of reflection symmetry. This is the situation we encountered earlier on in this chapter with the patchwork of hidden colours, because the inside-outside axis acted symmetrically with respect to the other two axes (dorsal-ventral and head-tail).
Fig. 14.8 Objects with a left-right axis can come in two possible forms and have no internal plane of reflection symmetry; whereas objects with bilateral symmetry have a single plane of reflection symmetry and come in only one form.
For objects with left-right asymmetry we can ask whether the two possible forms, L and R, have the same chance of occurring, or whether there is a bias that favours one over the other. Take the case of van Gogh cutting his ear. Was is just a matter of chance that he chose to cut his left rather than his right ear? Probably not. Based on a portrait of van Gogh at work by his friend Paul Gaugin, we can be fairly confident that van Gogh was right-handed. Now it seems to me that if a right-handed person wants to cut a bit of his ear off, he would most naturally choose his left ear: this ear is much easier for him to cut with the right hand while holding it with the left hand. There would therefore have been a bias that favoured one form over the other because of the handedness of van Gogh.
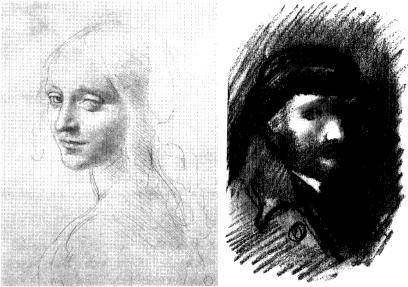
Such a bias arising from the handedness of artists can be witnessed in other ways. Compare the two sketches shown in Fig. 14.9, by van Gogh and Leonardo. Van Gogh's main lines of shading run diagonally from the top right of the picture to the bottom left, the way a right-handed person would naturally tend to shade things. By contrast, Leonardo, who was left-handed, naturally shaded in the opposite direction, from top left to bottom right. The direction of shading is not random but is biased by the preferred hand of the artist. We might call van Gogh's diagonals representative of the R-form of shading whereas Leonardo's would be typical of the L-form.
Fig. 14.9 Sketches by Leonardo (left) and van Gogh (right) showing opposite directions of shading.
For any individual artist there will therefore be a bias towards producing certain forms over others because of his or her hand preference. If you were to consider the works of many artists together, this bias might to some extent be averaged out because some are right-handed and others are left-handed. But the key point about handedness is that there is not an equal proportion of each type in human populations: there are more right-handed than left-handed people (I shall come back to why later). This means that there will be an overall bias towards certain forms, such as the direction of shading, over others. Our own handedness therefore provides a very good example of something that creates a bias towards one mirror image over the other. From now on I shall use handedness as a general term for any system in which there is a consistent bias towards an L- or an R-form.
Left-right asymmetry in plants and animals
There are many examples of left-right asymmetry in the living world. In some cases, the L- and the R-forms occur in equal numbers, and it is largely a matter of chance whether any individual will develop as one form or the other (i.e. there is no overall handedness). Male fiddler crabs, for example, often have an enlarged claw on one side (Fig. 14.10). Whether this is on the left (L-form) or right (R-form) depends on the previous chance experiences of the animal. Young males start off with two large claws, but if one of these is lost through injury, a small claw will regenerate in its place, resulting in a crab with a large claw on only one side. Unlike for van Gogh,

in crabs there seems to be no bias as to which side gets injured, so the left and right forms typically occur in equal numbers. (If both claws are lost, the crab ends up with two small claws; whereas if neither is lost, the adult ends up with a large claw on each side.)
Fig. 14.10 Male fiddler crab with enlarged left claw
Another example of chance asymmetry is the arrangement of leaves in some plants. For example, if you trace the consecutive leaves of a tobacco plant up the stem, they form a spiral or helix. The direction of the spiral can be most easily seen by looking down on the growing tip, from where the leaves further down appear progressively larger (Fig. 14.11). In some individual plants, the spiral runs clockwise from top to bottom (R-form), whereas in others it runs anticlockwise (L-form). These two forms are mirror images of each other. In a survey of over twenty thousand individual tobacco plants, the two forms were found to occur in approximately equal numbers. Furthermore, if either form was self-pollinated, it gave progeny which again were a 50:50 mixture of each type. It seems, then, to be largely a matter of chance whether an individual develops as one form or the other. Perhaps the direction that the plant will take is very finely balanced early on in development and a minor random perturbation is enough to flip it one way or the other.
Fig. 14.11 Two tobacco plants viewed from the top showing spiral arrangement of leaves.
In these examples, the distinction between left and right forms is a matter of chance. But there are other cases in which there is a consistent bias towards one form over the other. In flowers of greater periwinkle (Vinca major), for example, the petals are arranged as fan blades that all

seem to turn in the same way (Fig. 14.12, left). If you start from the centre of the flower and trace the edge of a petal outwards, you will be turning anticlockwise (the flower has five-fold rotational symmetry but no internal planes of reflection symmetry). This is a case of handedness because all individuals of this species have flowers arranged in the same manner (the L-form). By contrast, plants of oleander (Nerium oleander) have flowers that consistently exhibit the mirror image arrangement or R-form (Fig. 14.12, right).
Fig. 14.12Flowers of greater periwinkle (Vinca major) and oleander (Nerium oleander) showing handedness
Vertebrates typically exhibit bilateral symmetry on the outside, but several aspects of their internal anatomy show handedness. Our heart and stomach are usually on the left and our liver is on the right. This difference between internal and external asymmetry probably has to do with the way we move. Walking from A to B in a straight line is faster than moving in a left-handed curve, as would tend to happen if your left leg was shorter than your right. The only time we move in consistent curves is in the womb, where the foetus usually turns to its right, giving the vessels of the umbilical cord a corresponding twist (Fig. 14.13). Our external bodies are symmetrical for the same sort of reason that cars are outwardly symmetrical. Internally, though, we can put the steering wheel on the left or right of a car without influencing the direction it moves in. Similarly, we could argue that in order to pack our insides effectively, some organs need to go to one side or other. It is important that this is done in a consistent way. It is no good putting the steering wheel on the right of a car if the accelerator pedal is on the left. For similar reasons, individuals in which one or two organs are inverted from left to right while the other organs remain normal may have medical complications. About one in ten thousand people have all of their internal organs inverted, a mirror image of the normal arrangement, a condition termed situs inversus. Nevertheless, because the organs can still function properly with respect to each other, these individuals can live quite happily.
Fig. 14.13 Human umbilical cord showing twisted arrangement of blood vessels.
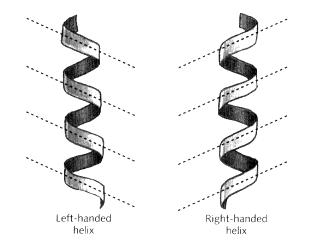
Although moving in left or right curves is not very common in animals, it is precisely the way that twining plants grow. When a twining plant climbs up a pole, the growing tip tends to curve consistently either to the right or left, resulting in a spiral or helix. I should briefly mention how the direction of a helix is defined. Helices are conventionally classified as either right-handed (R-form) or left-handed (L-form), according to whether or not they match the appearance of a right-handed corkscrew (Fig. 14.14). An easy way to recognise these forms is to look at the diagonal pattern made by the edges nearest to you. If they slope the same way as the shading of a right-handed person, the helix is right-handed; if they match the shading of a left-hander, the helix is left-handed (if you apply this to the umbilical cord shown in Fig. 14.13 you should easily see that it forms a left-handed helix). Note that whether the helix is right or left-handed is intrinsic to a helix and doesn't depend on which way up it is. You can see this by turning Fig. 14.14 upside down and observing that the direction of the diagonals remains unchanged (helices have rotational symmetry even though they have no internal plane of reflection symmetry).
Fig. 14.14 Two types of helix: left-handed and right-handed. Note how the diagonals of the helices slope in the same way as the shading of a person with the corresponding handedness.
Most species of twining plant show handedness, either consistently forming rightor left-handed helices when they climb. Runner beans (Phaseolus) and bindweeds (Convolvulus), for example, make right-handed helices, whereas hops (Humulus) and honeysuckles (Lonicera) produce left-handed helices (Fig. 14.15). The handedness depends on the behaviour of the growing tip. The tip of a twining plant tends to rotate once every few hours in a consistent direction as it grows, either clockwise or anticlockwise. It is the direction of this rotation that determines whether the plant will form a rightor left-handed helix as it climbs (Darwin made a fascinating study of this type of rotation, called circumnutation, by closely observing the tip of a pored plant in his room while he was confined there by illness).
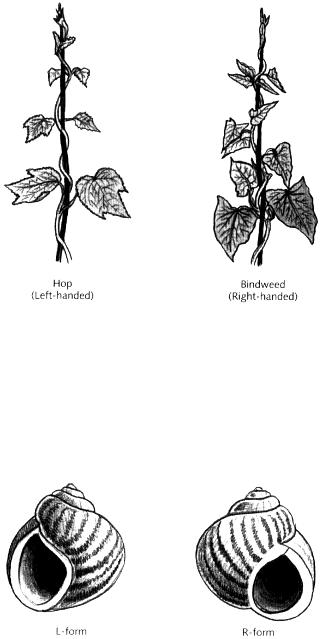
Fig. 14.15 Helices made by hop (left-handed) and bindweed (right-handed).
Another common example of handedness involving spiral growth is the direction of coiling in shells. Most snail or sea shells have right-handed spirals. If you look into such a shell, while holding it with the tip of the spiral pointing away from you, the opening is on the right (Fig. 14.16, right). The direction of the spiral depends on how the animal grows from a very early stage. In some right-handed species, rare mutant individuals can be found that grow in the opposite way and give left-handed shells.
Fig. 14.16 Left-handed and right-handed snail shells.
In all these cases of handedness, it is not at all clear, from the viewpoint of adaptation, why one mirror image should be favoured over the other. There is no obvious disadvantage in having all our internal organs arranged in the precise mirror image of the common form. Similarly, why should a twining plant or shell consistently spiral one way rather than the other? The answer is probably not that one mirror image works better than the other but that the basic molecular mechanisms that orient left-right asymmetries often happen to do so in a handed or biased manner. I now want to look at what these mechanisms might be.
The worm's turn
Handedness is challenging to explain because there has to be some way to orient the left-right
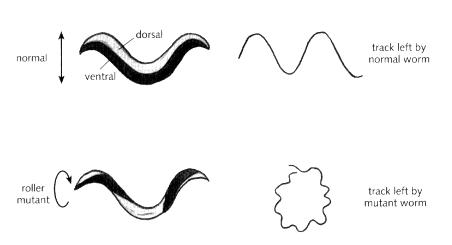
axis relative to the other axes in a consistent manner. As children it takes us a long time to distinguish left from right, so how can mere molecules or cells achieve this? We still do not know the definitive answer to this problem, but we can get a due by looking at some mutant worms.
The tiny nematode worm (Caenorhabditis elegans) normally moves along a solid surface by lying on its left or right side and undulating its body by a series of muscular contractions, leaving a wavy track (Fig. 14.17). However, mutant worms have been found that, instead of snaking smoothly along, roll around and move in circles. These roller mutants are twisted along their length so that their dorsal and ventral sides are helically arranged (Fig. 14.17). When such a mutant attempts to move, the head and body rotate like a screw, and the animal tends to travel in a circular path. Of most interest for our present purposes, roller mutants tend to circle around in a constant direction, showing handedness. There are two classes of roller mutant: those that circle clockwise and those that go anticlockwise. For each class, the worm behaves in a consistent manner. How is it that a mutation in a single gene can make a worm always turn one way?
Fig. 14.17 Movement and structure of a normal worm compared to a roller mutant.
It turns out that the gene affected in the roller mutants codes for a protein called collagen. Collagen is a long stiff protein that forms a major part of the hardened cuticle or outer covering that protects the worm (collagen is also found in humans, where it contributes the major fibrous element of skin and bone). A key feature of collagen proteins is that they are formed of three long twisted fibres that are always arranged as right-handed helices (Fig. 14.18). Now imagine that many of these collagen proteins were wrapped around the worm in parallel with each other. Because they all twist in the same way, their twists might well be compounded to give the covering of the worm an overall twist as well. And because the collagen proteins are handed, the overall twist would be biased predictably in one way or the other. In normal worms, this potential twisting may be averted by ensuring that the various collagen molecules (and other handed fibres) are oriented in particular ways with respect to each other. In other words, things are normally carefully arranged in order to avoid an overall twist. This means that a mutation that alters one type of collagen protein could disturb the balance, giving an overall rightor left-handed twist to the worm. The worm would then move in endless circles, clockwise or anticlockwise depending on the hand of the twist. This appears to be the sort of thing that happens in roller mutants. The consistent circular movement of mutant worms in one direction or another seems to derive from the handedness of its component collagen proteins. Why then are collagen proteins always