
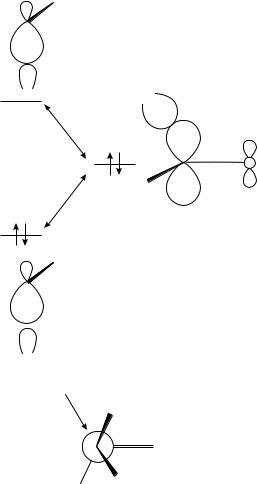
18. Nucleophilic attack on compounds containing CDC, CDO or CDN groups 1123
electron-donating substituent, D, will take up a position between the attacking nucleophile and the double-bond substituent to minimize electron donation into the system.
In terms of frontier orbitals, when the electron-withdrawing group is anti to the attacking nucleophile, its LUMO CŁ A overlaps with the HOMO of the transition state resulting
in stabilization, as shown in Figure 1042. The HOMO of the transition state is a mixture of the nucleophile HOMO and the carbonyl LUMO ( Ł ). If the electron-withdrawing group were in either of the other two staggered positions, the stabilization would be less because the overlap decreases the more the electron-withdrawing group is orthogonal to the direction of attack. Overlap of the occupied C D orbital of an electron-donating group with the HOMO of the transition state is destabilizing. Thus it prefers to take up a position orthogonal to the direction of attack and away from the double bond.
|
+ |
|
|
− |
|
|
+ |
|
σ |
A |
Nu |
|
||
|
+ |
|
|
|
ΗΟΜΟ
σ
+
−
−
D
Nu
Inside
+
−
+
−
Outside
Anti
FIGURE 10. Overlap of the LUMO CŁ A of an electron-withdrawing group on the ˛ carbon with the HOMO of the transition state. Reprinted with permission from Reference 42. Copyright (1986) American Association for the Advancement of Science

1124 |
Peter G. Taylor |
Of course, sometimes steric factors and electronic factors may compete. For example, if the electronic effects of the trimethylsilyl substituent dominate, it will take up a position opposite the carbonyl; however, if its steric factors dominate, it will take up a position anti to the incoming nucleophile. Thus one of the problems with this model, with some systems, is determining which group should be placed antiperiplanar to the attacking nucleophile, since its selection depends upon both steric factors and electronic factors. Wong and Paddon-Row have used ab initio calculations to model the complete set of diastereoisomeric transition states for the addition of cyanide to propanal, fluoroethanal and 2-fluoropropanal71. They found that the most stable transition state has the C F bond antiperiplanar to the forming Nu C bond, consistent with the Anh Eisenstein model. Their results were not consistent with the alternative Cieplak model72, which suggests mixing of the vacant Ł orbital of the forming nucleophile bond with the filled orbital of the C L bond is important. Electrostatic effects have also been shown to be important in the nucleophilic addition to ˇ, -unsaturated carbonyl compounds73, which give high stereoselectivity74. In non-chelated systems the transition state has the alkynyl group anti to the incoming nucleophile, not because of its size (it has little steric demand) but because of electronic effects. Calculations indicate that in the presence of a metal ion and chelation, the alkynyl group occupies the ‘inside’ position.
Another empirical model is Prelog’s rule for predicting the stereoselectivity of nucle-
ophilic attack at the carbonyl of an ˛-ketoester of a chiral alcohol, RCOCO2RŁ75,76 . The two carbonyls are arranged anti and the larger of the substituents on the chiral centre is arranged so that it is anti to the O C bond. Attack of the nucleophile on the carbonyl then occurs from the side with the smallest of the other two groups attached to the chiral centre (Scheme 14). Ab initio calculation combined with molecular mechanics calculations42 have confirmed that the conformation with the largest group anti to the O C bond is preferred and this anchors the positions of the small and medium groups, such that they control the facial selectivity.
O
L O
Nucleophile attacks from above
M S O
SCHEME 14
C. Stereoselectivity of Nucleophilic Attack on Cyclic Ketones
It is found experimentally that cyclohexanones undergo preferential axial attack with nucleophiles. Klein has explained this preference in terms of the frontier orbitals79. The orbital is distorted about the CDO plane such that the LUMO has a big lobe on the axial side and the HOMO has a big lobe on the equatorial side. Thus for good overlap of the attacking nucleophile HOMO with the carbonyl LUMO, axial attack is preferred. This also reduces the destabilizing interaction of the nucleophile HOMO with the carbonyl HOMO. Shi and Boyd have studied the valence-shell charge concentration of the carbonyl in cyclohexanones and found that the charge density changes only slightly with substituents and is similar on the two sides of the carbonyl80. If anything the model predicts the wrong stereoselectivity, thus they conclude that the difference in the extent of electron deficiency between the two sides of the carbonyl does not play a significant role in stereoselectivity.
Anh69,81 has suggested that the interaction of the incipient bonding orbital with the antiperiplanar bond’s antibonding orbital affects the relative energy of the transition states,

18. Nucleophilic attack on compounds containing CDC, CDO or CDN groups 1125
such that when there is good overlap the transition state is stabilized. Cieplak72,82 has suggested that the stability of the incipient antibonding orbital is affected by the electrondonating ability of neighbouring bonds. For axial attack the neighbouring bonds are the axial bonds at C(2) and C(6). For equatorial attack the neighbouring bonds are the carbon carbon bonds C(2) C(3) and C(5) C(6). The preferred direction of attack is thus determined by the relative electron-donating ability of these bonds. He proposed that the electron-donating ability of various bonds follows the order C S > C H > C C > C N > C O. Thus with cyclohexanone the C H bonds are more electron-donating than the C C bonds, thus axial attack predominates. Le Noble’s group examined the nucleophilic attack of 5-substituted adamantones to eliminate steric effects83 (Scheme 15).
O |
|
Nu |
OH |
|
Nu− |
OH |
Nu |
|
|
+ |
|
|
|
|
|
R |
|
R |
R |
SCHEME 15
Electron-donating groups in the 5 position led to mainly anti attack, in agreement with Cieplak’s proposal, since attack is anti to the electron-rich carbon carbon bonds. When electron-withdrawing groups were used, mainly syn attack was observed as expected. Coxon and McDonald have used molecular orbital calculations to examine the facial selectivity of a series of 7-norbornanones with C(2) and C(3) endo substituents which exhibit electronic effects on the reaction centre, but, because of the rigid nature of the framework, they do not alter the steric environment in the region of the reaction centre84. (Scheme 16). They found that attack of methanol led to a transition state where the forming oxygen carbon bond is substantially made. The bonds antiperiplanar to the incoming oxygen are little changed, but those antiperiplanar to the carbonyl oxygen are shortened. The presence of electron-withdrawing substituents at C(2) and C(3) leads to shorter bonds anti to the C(7) OMe bond in the transition state. However, electron-donating alkyl groups at C(2) and C(3) have little effect on these bonds. The authors conclude that the Cieplak postulate is not supported by their semi-empirical calculations. Earlier, Mehta had experimentally determined the selectivities for nucleophilic attack of borohydride on 7-norbornanones. The experimental results and those of theoretical calculations are shown in Table 285. The results for the carbomethoxy group were thought to differ because of the difference in the nature of the two nucleophiles: one is charged and the other neutral.
O
anti |
|
|
|
|
|
syn |
|
|
|
R
R
SCHEME 16
Shi and Boyd80 have studied the barriers to nucleophilic addition of lithium hydride to substituted cyclohexanones. They found that the unsubstituted cyclohexanone had a

1126 |
|
Peter G. Taylor |
|
||
|
TABLE 2. Observed and calculated syn:anti |
ratios for nucleophilic |
|||
|
attack on 7-norbornanones |
|
|||
|
|
|
|
|
|
|
Substituent R |
Experimental syn:anti |
Calculated syn:anti |
||
|
|
ratio |
ratio |
||
|
|
|
|
|
|
|
H |
50:50 |
50:50 |
||
|
Ethyl |
20:80 |
33:67 |
||
|
CO2CH3 |
84:16 |
7:93 |
||
|
F |
|
|
|
7:93 |
|
|
|
|
||
|
|
|
|
|
|
larger barrier to attack than the substituted counterparts as observed experimentally86. Secondly, the difference between the barriers to axial and equatorial attack was largest for 4-substituted cyclohexanones and that whilst the barrier to axial attack showed little variation with position of the substituent, the barrier to equatorial attack did vary significantly with the position of the substituent, being particularly large when the substituent was axial. They calculated the electron-donating ability of the appropriate bonds for the series of substituted cyclohexanones to see if the pattern matched that predicted by Cieplak and found significant discrepancies.
Shi and Boyd suggest that the difference between the selectivity of 4-equatorially- substituted cyclohexanones and 4-axially-substituted cyclohexanones arises from the change in the direction of the dipole orthogonal to the carbonyl plane. This is consistent with Kamernitzky and Akhrem’s proposal that the facial selectivity is ‘determined by a difference in the electrostatic fields on the upper and lower sides of the carbonyl double bond’87.
Wipf has shown that 4,4-disubstituted cyclohexanones undergo nucleophilic attack where the facial selectivity is determined by dipolar control. Thus, compounds of the type 23 underwent nucleophilic attack anti to the electronegative substituent at C(4), whereas the fluorinated analogue, 24, underwent attack syn to the oxygen, in accordance with the inversion of the dipole moment. They found that the logarithm of the experimentally observed facial selectivity for nucleophilic attack was correlated linearly (R D 0.998) with the calculated dipole moments. The facial selectivities were also shown to depend upon the nature of the nucleophile, hydride ions and alkynyl carbanions being essentially unselective.
O O
Me OMe |
CF3 CF2 OMe |
(23) |
(24) |
The preferred direction of nucleophilic attack on cyclohexanones has also been explained in terms of the torsional strain between the forming carbon nucleophile bond and the adjacent bonds on C(2) and C(6)66,68,81 (Scheme 17). If the ring is flat, attack from the axial side is staggered whereas equatorial attack is eclipsed. However, if the ring is puckered, attack from the axial side is eclipsed and the equatorial side is staggered. Shi and Boyd have confirmed with calculations at the 6-21G or 6-32G level that the flatter the ring the more axial attack80.

18. Nucleophilic attack on compounds containing CDC, CDO or CDN groups 1127
axialattack |
|
|
α |
β |
O |
|
equatorial attack
SCHEME 17
One of the problems of modelling the stereochemistry of nucleophilic addition to carbonyls is predicting the influence of metal cations. Some simple force field calculations88 and steric congestion models89 have been successful in accounting for the stereoselectivities of hydride reductions of cyclic ketones. Molecular mechanics calculations ignoring the metal ion but using a suitably reduced have been successful in explaining the stereoselectivity of LiAlH4 reduction of cyclobutanone and cyclohexanones42.
D. Diastereoselectivity as a Result of a Chiral Centre b to a Carbon Double Bond in the Substrate
The presence of a chiral centre ˇ to a carbonyl also provides the possibility for chirality transfer upon nucleophilic attack. In cases where there is the possibility of forming an internal chelate, 25, the stereochemistry is explained by attack of the carbonyl opposite the bulky R2 group. However, when chelation is not possible, the group of Reetz has suggested that a transition state such as 26 is involved when the chiral carbon has a polar X group which points away from the carbonyl and the nucleophile attacks from the face opposite the bulky R group77.
|
|
|
H |
|
|
|
R |
|
|
M |
R1 |
|
|
|
|
||
|
|
|
|
|
|
C X |
||
O |
O |
− |
+ |
|
|
|
||
|
|
|
||||||
F3 B |
|
O |
|
C H |
||||
|
||||||||
|
|
|||||||
|
||||||||
|
|
H |
|
|
|
|
|
H |
|
|
|
|
|
|
|
|
|
H |
|
R2 |
H |
|
|
Nu: |
||
|
(25) |
|
|
|
(26) |
|
|
Evans and coworkers have proposed an alternative model for 1,3-asymmetric induction for nucleophilic additions to aldehydes bearing polar substituents in the ˇ position78. The carbonyl is orientated at right angles to the C(˛) C(ˇ) bond in accord with the Felkin assertion that such a staggered arrangement is preferred. Minimization of the interacting dipoles and non-bonded interactions gives the most stable transition state, 27, with nucleophilic attack of the top face of the carbonyl. Other transition states, such as 28 and 29, suffer from destabilizing gauche interactions of R and unfavourable interactions of
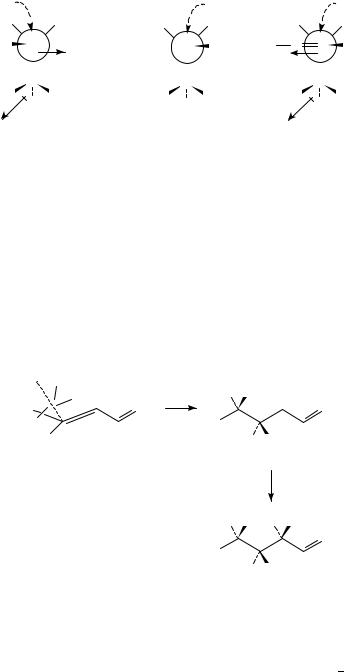
1128 |
|
|
|
|
|
|
Peter G. Taylor |
|
|
|
||||||
Nu: |
|
|
|
|
|
|
|
|
|
|
|
|
Nu: |
|
|
Nu: |
H2 |
|
|
|
H1 |
|
H2 |
H1 |
H2 |
|
H1 |
||||||
H C |
|
O |
|
M |
M |
|
O |
|
C H |
M O C H |
||||||
|
|
|||||||||||||||
|
|
|
|
|||||||||||||
|
+ |
|
|
|
|
|
|
|
|
|
|
|
|
+ |
||
|
|
|
|
|
|
|
|
|
|
|
|
|
|
|
|
|
|
C |
|
|
|
C |
|
|
C |
||||||||
X |
|
|
H |
|
|
H |
|
R |
X |
|
H |
|||||
|
R |
|
|
|
X |
|
|
R |
||||||||
|
(27) |
|
|
(28) |
|
|
(29) |
the two dipoles, respectively. From this model it follows that the selectivity should be enhanced with an increase in the steric bulk of R, as was observed.
E. Stereoselectivity of Conjugate Additions
The stereochemistry of conjugate additions requires similar considerations to those used to predict nucleophilic attack on a carbonyl. Acyclic substrates may yield up to three contiguous chiral centres (Scheme 18). The relative stereochemistry of carbons C(1) and C(2) will depend upon the approach of the two reagents. The stereochemistry of carbon C(3) will depend upon the lifetime of the intermediate 30; if it has even a short lifetime it will take up the most stable conformation. For example, the base-catalysed additions of ethanol-d and 2-methyl-2-propanethiol-d to ethyl crotonate give a (2RŁ , 3RŁ / 2RŁ , 3SŁ) diastereoisomeric ratio of the addition products of approximately 10:190. The authors90 suggest the reaction proceeds in two steps and the protonation of the enolate determines the stereoselectivity.
|
M |
|
M L |
|
|
C − |
L |
|
|
L |
− |
X |
||
|
X |
|
||
S |
|
|
S |
|
|
S |
|
L S |
|
|
|
|
|
|
|
|
|
(30) |
|
|
M L |
|
H E |
|
|
|
2 |
X |
|
S |
1 |
3 |
||
|
||||
|
|
|
||
|
L |
|
S |
SCHEME 18
The conjugate addition of hydrazine, hydroxylamine, methoxylamine and alkylamines with various alkyl and halegenofumaric acids to give N-substituted aspartic acids can be carried out enantioselectively in the presence of the enzyme 3-methylaspartase91.
Chiral centres within the nucleophile or the conjugated double bond will control the stereochemistry of the chiral centres that are formed. For example, the Felkin Ahn model has been applied to nucleophilic 1,4-addition to an ˛,ˇ-unsaturated carbonyl bearing a
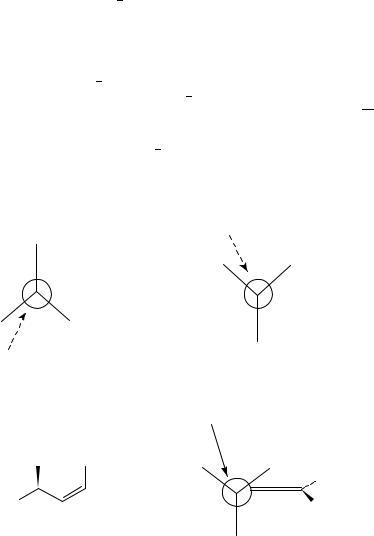
18. Nucleophilic attack on compounds containing CDC, CDO or CDN groups 1129
-stereocentre. When the carbon carbon double bond is trans, the outside position is the most crowded because of the incoming nucleophile. Hence this position is occupied by the smaller of the groups on the chiral centre. The medium-sized group thus occupies the inside position and the large group directs attack to the opposite face. Whilst such behaviour is often observed92,93, this is not always the case.
Dorigo and Morokuma have highlighted that the main problem concerned with the ‘homologation’ of the Felkin Ahn model to 1,4-additions is identifying the preferred position of the medium-sized group with cis carbon carbon double bonds94. Does the presence of the cis substituent make the inside or the outside positions the most crowded does 31 or 32 resemble the transition state? Both models have been used to rationalize the conflicting evidence. For example, nucleophilic attack of the (Z)- -methoxy ˛,ˇ-unsaturated esters (Scheme 19) follows the Felkin Anh model with the C OMe bond antiperiplanar to the direction of the nucleophilic attack. The larger methyl group occupies the ‘outside’ position and the hydrogen the ‘inside’ position, thus avoiding any 1,3- allylic strain95.
|
|
anti |
|
|
|
|
|
|
|
|
|
Nu |
|
|
|
|
|
|
|
|
||
|
|
L |
|
|
|
|
|
|
|
|
|
|
|
|
|
|
|
|
|
|
|
|
|
|
|
|
|
|
|
|
|
|
|
|
|
M |
|
S |
|||||||
H |
|
|
|
|
C |
|
C |
|
O |
|
H |
|
|
|
|
C |
|
C |
|
O |
||
|
|
|
|
|
|
|
||||||||||||||||
|
|
|
|
|
||||||||||||||||||
|
|
|
|
|
|
|
||||||||||||||||
|
|
|
|
|
|
|
|
|
|
|
|
|
|
|
|
|
|
|
|
|
|
|
|
S |
|
|
|
R |
|
|
|
|
|
|
|
|
|
|
R |
||||||
|
M |
|
|
|
|
|
|
|
|
|
|
|
|
|
|
|||||||
outside |
inside |
|
|
|
|
|
|
L |
||||||||||||||
|
|
|
|
|
|
|
|
|
|
|
|
|
|
|
||||||||
|
Nu |
|
|
|
|
|
|
|
|
|
|
|
|
|
|
|
|
|
|
|
||
(31) |
|
|
|
|
|
|
|
|
|
|
(32) |
|
|
|
|
|
|
|||||
|
|
|
|
|
|
|
|
|
|
|
|
Nuc |
|
|
|
|
|
|
|
|
||
|
|
|
|
CO2 Me |
|
‘outside’ |
|
‘inside’ |
||||||||||||||
|
|
OMe |
|
|
|
Me |
H |
|||||||||||||||
|
|
|
|
|
|
|
|
|
|
|
H |
|
|
|
|
H |
||||||
|
Me |
|
|
|
|
|
|
|
|
|
|
CO2 Me |
||||||||||
|
|
|
|
|
|
|
|
|
|
|||||||||||||
|
|
|
|
|
|
|
|
|
|
|
|
|
||||||||||
|
|
|
|
|
|
|
|
|
|
|
|
|
|
|
|
OMe
SCHEME 19
Molecular mechanics calculations96 and ab initio studies94 have been used to resolve the problem, but whilst they go some way to explaining the stereochemical outcomes, there are a number of reactions whose stereochemistry cannot be easily explained.
Enantiomerically pure vinyl sulphoxides are highly diastereoselective Michael acceptors with a variety of nucleophiles97. However, the transition states that determine the stereochemical outcome are not easy to predict. For example, the nucleophilic attack of piperidine on ( )-(R)-(Z)-propenyl p-tolyl sulphoxide98 gives a product that can be explained by approach of the nucleophile from the side of the tolyl group, away from
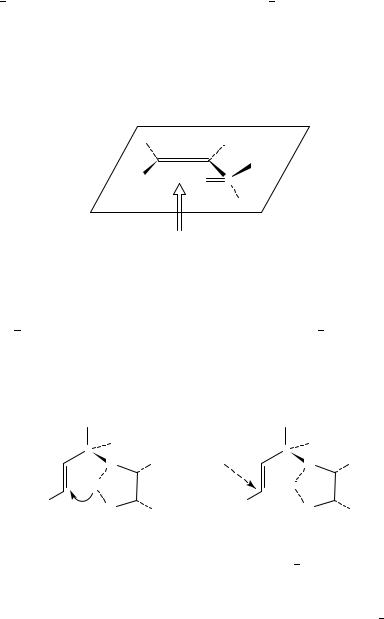
1130 |
Peter G. Taylor |
the lone pair, when the SDO and CDC bonds are cis coplanar, or by approach of the nucleophile from the side of the oxygen when the lone pair eclipses the double bond. Kahn and Hehre have calculated that the most stable conformation is that with the sulphur oxygen double bond cis coplanar with the carbon carbon double bond99. They predict that hydride ion will attack from the side of the methyl group, contrary to steric considerations, but consistent with the nucleophile avoiding areas of high electron density (Scheme 20). They suggest that examples that are not consistent with this approach may involve ion pairing between the lone pairs and a metal ion which reduces the unfavourable interaction between the lone pair and the incoming nucleophile. The stereochemistry of intramolecular nucleophilic attack on vinyl sulphones is controlled by the stereochemistry of the sulphone and/or any other stereogenic centres in the molecule100.
H H
H O S
CH3
Nucleophile attacks from below
SCHEME 20
Vinyl sulphoximines also readily undergo stereoselective nucleophilic attack. In this case the nitrogen on the sulphur can coordinate any metals and direct attack101,102. The sulphur oxygen bond is thought to be anti coplanar with the carbon carbon double bond and, if the carbanion is associated with the complexed metal, attack from the same side as the nitrogen, 33, is preferred. If a metal ion is complexed with the nitrogen but the carbanion is not associated with this metal ion, 34, attack occurs from the opposite side to the nitrogen.
|
O |
|
|
O |
|
|
Ph |
|
|
Ph |
|
|
S |
Me |
RM |
S |
Me |
|
N |
N |
|||
|
RM |
|
|
Li |
|
Ph |
MeO |
Ph |
Ph |
MeO |
Ph |
|
|
||||
|
|
|
|
||
|
(33) |
|
|
(34) |
|
The nitro group in 35 (Scheme 21) activates the carbon carbon double bond to nucleophilic attack. In this case the presence of the adjacent chiral centre led to predominantly one diastereoisomer103. The results suggested that in this case 1,3-allylic strain is not important and attack predominantly took place on the conformer with the phenyl occupying the ‘inside’ position, possibly because of the longer carbon sulphur bond distance, and the hydrogen occupying the ‘outside’ position, allowing the nucleophile to approach the nitroalkene in close proximity to the hydrogen. A similar overwhelming

18. Nucleophilic attack on compounds containing CDC, CDO or CDN groups 1131
Nuc |
|
|
H |
TBSO |
O |
Ph |
|
|
H |
NO2 |
|
Ph |
SPh |
|
SPh |
|
Nuc |
OTBS |
TBS = tert - butyldimethylsilyl |
|
(35)
of the 1,3-allylic strain by factors elsewhere in the molecule has been observed for attack on other nitroalkenes93,104.
V.REFERENCES
1.S. Yamabe, T. Minato and Y. Kawabata, Can. J. Chem., 62, 235 (1984).
2.O. I. Asubiojo and J. I. Brauman, J. Am. Chem. Soc., 101, 3715 (1979).
3.C. M. Evans and A. J. Kirby, J. Chem. Soc., Perkin Trans. 2, 1259 (1984).
4.C. M. Evans and A. J. Kirby, J. Chem. Soc., Perkin Trans. 2, 1269 (1984).
5.G. M. R. Tombo, R. A. Pfund and C. Ganter, Helv. Chim. Acta, 64, 813 (1981).
6.Z. Rappoport, Acc. Chem. Res., 14, 7 (1981).
7.Z. Rappoport, Acc. Chem. Res., 25, 474 (1992).
8.See, for example, Z. Rappoport, in Adv. Chem. Ser. (215): Nucleophilicity (Eds. J. M. Harris and S. P. McManus), 1987.
9.See, for example, C. F. Bernasconi, in Adv. Chem. Ser. (215): Nucleophilicity (Eds. J. M. Harris and S. P. McManus), 1987.
10.H. Shenhav, Z. Rappoport and S. Patai, J. Chem. Soc. (B), 469 (1970).
11.R. Connor and W. R. McClellan, J. Org. Chem., 3, 570 (1938); E. D. Bergmann, D. Ginsburg and R. Pappo, Org. React., 10, 179 (1959).
12.C. K. M. Heo and J. W. Bunting, J. Org. Chem., 57, 3570 (1992).
13.M. Friedman and J. S. Wall, J. Org. Chem., 31, 2888 (1966).
14.T. L. Amyes and A. J. Kirby, J. Am. Chem. Soc., 110, 6505 (1988).
15.J. L. Bada and S. L. Miller, J. Am. Chem. Soc., 91, 3948 (1969).
16.M. T. Carroll, J. R. Cheeseman, R. Osman and H. Weinstein, J. Phys. Chem., 93, 5120 (1989).
17.L. Pardo, R. Osman, H. Weinstein and J. Rabinowitz, J. Am. Chem. Soc., 115, 8263 (1993).
18.R. G. Parr and W. Yang, J. Am. Chem. Soc., 106, 4049 (1984).
19.W. Langenaeker, K. Demel and P. Geerlings, J. Mol. Struct. (Theochem.), 259, 317 (1992).
20.R. M. Magid, Tetrahedron, 36, 1901 (1980).
21.L. A. Paquette and C. J. M. Stirling, Tetrahedron, 48, 7383 (1992).
22.F. G. Bordwell, Acc. Chem. Res., 3, 281 (1970); F. G. Bordwell and T. G. Mecca, J. Am. Chem. Soc., 94, 5829 (1972).
23.See, for example, Reference 21, p. 7395.
24.D. J. McLennan, Acc. Chem. Res., 9, 281 (1976); H. Meislich and S. J. Jasne, J. Org. Chem., 47, 2517 (1982).
25.See, for example, G. Stork and W. N. White, J. Am. Chem. Soc., 78, 4609 (1956); R. M. Magid and O. S. Fruchey, J. Am. Chem. Soc., 101, 2107 (1979).
26.G. Stork and A. R. Schoofs, J. Am. Chem. Soc., 101, 5081 (1979).
27.K. Fukui, Acc. Chem. Res., 4, 57 (1971).
28.N. D. Epiotis, W. R. Cherry, S. Shaik, R. L. Yates and F. Bernardi, Top. Curr. Chem., 70, 1 (1977).
29. G. Quinkert, H.-G. Schmalz, E. Walzer, S. Gross, T. Kowalezyk-Przewloka, G. Schierloh,
G. Durner, J. W. Bats and H. Kessler, Justus Liebigs Ann. Chem., 283 (1988).
30.J. F. King, J. H. Hillhouse and S. Skonieczny, Can. J. Chem., 62, 1977 (1984).
31. R. D. Chambers, in Synthetic Fluorine Chemistry (Eds. G. A. Olah, R. D. Chambers and G. K. S. Prakash), Wiley, New York, 1992.
1132 |
Peter G. Taylor |
32.M. R. Bryce, R. D. Chambers and G. Taylor, J. Chem. Soc., Perkin Trans. 1, 509 (1984).
33.M. W. Briscoe, R. D. Chambers, S. J. Mullins, T. Nakamura and F. G. Drakesmith, J. Chem. Soc., Chem. Commun., 1127 (1990).
34.R. D. Chambers and M. P. Greenhall, J. Chem. Soc., Chem. Commun., 1128 (1990).
35.G. Stork and B. Ganem, J. Am. Chem. Soc., 95, 6152 (1973).
36.M. P. Cooke Jr. and C. M. Pollock, J. Org. Chem., 58, 7474 (1993).
37.A. R. Bassindale and P. G. Taylor, in The Chemistry of Organosilicon Compounds (Eds. S. Patai and Z. Rappoport), Wiley, Chichester, 1989.
38.N. Auner, J. Prakt. Chem., 337, 79 (1995).
39.H. B. Burgi¨ and J. D. Dunitz, Acc. Chem. Res., 16, 153 (1983).
40.For a recent review, see; A. J. Kirby, Adv. Phys. Org. Chem., 29, 87 (1994).
41.P. Rademacher, Chem. Soc. Rev., 143 (1995).
42.K. N. Houk, M. N. Paddon-Row, N. G. Rondan, Y.-D. Wu, F. K. Brown, D. C. Spellmeyer,
J. T. Metz, Y. Li and R. J. Loncharich, Science, 231, 1108 (1986).
43.M. N. Paddon-Row, N. G. Rondan and K. N. Houk, J. Am. Chem. Soc., 104, 7162 (1982).
44.R. W. Strozier, P. Caramella and K. N. Houk, J. Am. Chem. Soc., 101, 1340 (1979).
45.E. Kaufmann, P. v. R. Schleyer, K. N. Houk and Y.-D. Wu, J. Am. Chem. Soc., 107, 5560 (1985).
46.K. N. Houk, N. G. Rondan, P. v. R. Schleyer, E. Kaufmann and T. Clark, J. Am. Chem. Soc., 101, 2821 (1979).
47.C. L. Liotta, E. M. Burgess and W. H. Eberhardt, J. Am. Chem. Soc., 106, 4849 (1984).
48.J. E. Baldwin, J. Chem. Soc., Chem. Commun., 734 (1976).
49.J. E. Baldwin, J. Cutting, W. Dupont, L. Kruse, L. Silberman and R. C. Thomas J. Chem. Soc., Chem. Commun., 736 (1976).
50.J. E. Baldwin and J. A. Reiss, J. Chem. Soc., Chem. Commun., 77 (1977).
51.J. E. Baldwin and L. I. Kruse, J. Chem. Soc., Chem. Commun., 233 (1977).
52.M. E. A. Astudillo, N. C. J. Chokotho, T. C. Jarvis, C. D. Johnson, C. C. Lewis and P. D. McDonnell, Tetrahedron, 41, 5919 (1985).
53.C. D. Johnson, Acc. Chem. Res., 26, 476 (1993).
54. G. W. Ellis, C. D. Johnson and D. N. Rogers, J. Chem. Soc., Chem. Commun., 36 (1982);
G. W. Ellis, C. D. Johnson and D. N. Rogers, J. Am. Chem. Soc., 105, 5090 (1983).
55.C. M. Brennan, I. Hunt, T. C. Jarvis, C. D. Johnson and P. D. McDonnell, Can. J. Chem., 68, 1780 (1990).
56.P. Metzner, Synthesis, 1185 (1992).
57.A. R. Bassindale, R. J. Ellis, J. C.-Y. Lau and P. G. Taylor, J. Chem. Soc., Chem. Commun., 98 (1986).
58.A. G. M. Barrett, J. M. Hill, E. M. Wallace and J. A. Flygare, Synlett., 765 1991.
59.D. Bell, E. A. Crowe, N. J. Dixon, G. R. Green, I. S. Mann and M. R. Skipton, Tetrahedron, 50, 6643 (1994).
60.See, for example, D. A. Evans, J. V. Nelson and T. R. Taber, Top. Stereochem., 1, 13 (1982).
61.H. Kunz and W. Pfrengle, Angew. Chem., Int. Ed. Engl., 28, 1067 (1989).
62.G. Solladie, in Stereochemistry, Fundamentals and Methods (Ed. H.B. Hagan), Vol. 3, Thieme, Stuttgart, 1977.
63.S. E. Denmark, N. Nakajima and O. J.-C. Nicaise, J. Am. Chem. Soc., 116, 8797 (1994).
64.E. L. Eliel, in Asymmetric Synthesis (Ed. J. D. Morrison), Vol. 2, Academic Press, London, 1983.
65.W. Cornforth, R. H. Cornforth and K. K. Mathews, J. Chem. Soc., 112 (1959).
66.M. Cherest, H. Felkin and N. Prudent, Tetrahedron Lett., 2199 (1968).
67.M. Cherest, H. Felkin and N. Prudent, Tetrahedron Lett., 2201 (1968).
68.M. Cherest and H. Felkin, Tetrahedron Lett., 2205 (1968).
69.N. T. Anh and O. Eisenstein, Nouv. J. Chem., 1, 61 (1977).
70.I. Fleming and J. J. Lewis, J. Chem. Soc., Chem. Commun., 149 (1985).
71.S. S. Wong and M. Paddon-Row, J. Chem. Soc., Chem. Commun., 456 (1990).
72.A. S. Cieplak, J. Am. Chem. Soc., 103, 4540 (1981).
73.M. Fujita, S. Akimoto and K. Ogura, Tetrahedron Lett., 34, 5139 (1993).
74.E. P. Lodge and C. H. Heathcock, J. Am. Chem. Soc., 109, 2819 (1987).
75.V. Prelog, Helv. Chim. Acta, 36, 308 (1953).
76.J. C. Fiaud, in Stereochemistry, Fundamentals and Methods (Ed. H. B. Hagan), Vol. 3, Thieme, Stuttgart, 1977.