
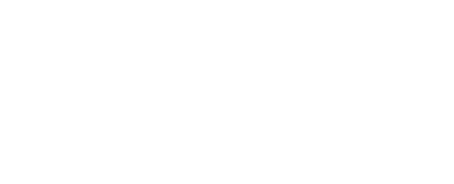
4. The chiroptical properties of the carbon |
|
carbon double bond |
147 |
|
FIGURE 9. The absorption ( |
|
) and CD (- - - - -) spectra of (R)-( )-(4-methylcyclohexylidene) |
|
||
ethane in the gas phase |
|
|
Unlike the olefins mentioned so far, the spectrum of 4-methylcyclohexylidene chromophore showed a rich structure, even at higher energies than the ! Ł transition. The transitions were assigned as belonging to higher Rydberg transitions. For example, for the ns Rydberg series, bands were detected with members up to n D 7. In Figure 9 we present the absorption and CD spectra of (4-methylcyclohexylidene)ethane in the gas phase. The second purpose of the study63 was to determine whether, and in what manner, a substituent lying in the nodal plane of the orbital contributed to the CD. Deuterium66,67 and fluorine68,69 substituents, which are known to exhibit an antioctant effect on the n ! Ł of carbonyls, were of special interest in our system, in which the molecular asymmetry was due to having both a substituent other than hydrogen in the 4-position of the cyclohexane ring and a situation in which X did not equal Y in Figure 8. Any contribution of allylic axial bond in Figure 8 would, of course, cancel each other out. In all our compounds the methyl substituent in the 4-position is kept constant and only the X and Y attached to the double bond are varied. It is therefore the effect of the X and Y substituents that will determine the sign of the ! Ł CD signal. In particular, we have explored the effect of the deuterium and fluorine atoms on the ! Ł transition. The results presenting the sign of the ! Ł transition for the R configuration are summarized in Table 3. It can be seen that, with the exception of deuterium, when Y D H a negative CD sign is observed for the ! Ł transition. The alkyl substituents, as well as the halogens, including fluorine, exhibited the same consignate effect. Attempts to correlate the sign of the CD spectra of the fluorine derivatives with mass or polarizability70 of the substituents failed. The results were rationalized in terms of the C X vs C Y bond lengths. When the covalent radius of X > Y, then the CD sign of the ! Ł transition will be negative, and when Y > X it will be positive, as long as the substituent X or Y does not possess a delocalizing system at the point of attachment to C. The dependence of the CD sign on RC X n, where RC X is the distance between the chromophore and a substituent X, is common to all three semiempirical mechanisms (static perturbation, dynamic coupling and the coupled oscillator71) explaining optical activity. These relations were raised in an attempt to account for the experimental results.
A more serious explanation was offered recently72. The independent systems perturbation (ISP) approach was used to calculate the CD of the ! 3s and ! Ł transitions. The ISP approach is interested in a particular transition belonging to an achiral chromophore A; the other chromophores in the molecule interact with this transition giving it

148 |
Aharon Gedanken |
TABLE 3. Configuration, atomic radii and sign of ! Ł CD of 1 substituted by various X and Y groups
Configuration |
Substituent (radii, A)˚ |
|
CD ( ! Ł ) |
||
|
X |
Y |
|
Predicted |
Found |
|
|
|
|
|
|
(R)-( )Ð3 |
Br (1.14) |
H (0.37) |
( ) |
( ) |
|
(R)-( )Ð4 |
CH3 (0.77) |
H (0.37) |
( ) |
( ) |
|
(R)-( )Ð5 |
CD3 (0.77) |
H (0.37) |
( ) |
( ) |
|
(R)-(C)Ð7 |
D (0.371) |
H (0.373) |
|
(C) |
(C) |
(R)-( )Ð8 |
Cl (0.99) |
H (0.37) |
( ) |
( ) |
|
(R)-( )Ð9 |
CH2CH3 (0.77) |
H (0.37) |
( ) |
( ) |
|
(R)-( )Ð6 |
t-Bu (0.77) |
H (0.37) |
( ) |
( ) |
|
(R)-( )Ð13 |
F (0.72) |
D (0.371) |
( ) |
( ) |
|
(R)-( )Ð14 |
F (0.72) |
H (0.373) |
( ) |
( ) |
|
(R)-( )Ð11 |
Br (1.14) |
F (0.72) |
( ) |
( ) |
|
(R)-( )Ð12 |
F (0.72) |
CH3 (0.77) |
|
(C) |
(C) |
a helical character. A perturbing chromophore that lies on the reflection plane of A gave on helical character. Two identical chromophores symmetrically placed with respect to a reflection plane have no net effect. Thus one might expect the ethylene chromophore transitions of Figure 9 to have no CD since the substituents lie on reflection planes of the chromophore framework. In fact, small CD were observed for these transitions as a result of the concerted coupling of both substituents to the transitions of A. The final explanation for the deuterium dissignate behavior is that it is a reflection of the fact that the C D bond length is less than the C H one.
A theoretical attempt that has provided the basis for the octant rule42 was developed by Scott and Yeh73. They have considered the intensity of the CD band from the ground state to an excited state as consisting of three components:
R D Rinherent C Rstatic C Rdynamic |
6 |
For olefins, the first term has been studied in terms of the symmetry D2 independently by two groups9,13. The second term, which was also called the one-electron term, is usually neglected in cases of electronically allowed transitions because it is small compared with the other two terms. It is even smaller in the olefinic case, where the molecules contain mainly nonpolar bonds. Scott and Yeh73 have therefore concentrated on the third term, which arises from the coupling of a considered transition a with all the transitions of different chromophores within the molecule. If transition a falls energetically below all the transitions of the other chromophores, the latter can be viewed as polarizable groups and their anisotropies ˇ determine the dynamic coupling74. The final expression obtained was
Rdynamic ! Ł D 208.7 ˇb b10 40 cgs Ð esu
where ˇ is the anisotropy for which three sets of values were introduced and tried in their73 calculations. The factor contained, besides, a directional factor, an expression that is inversely proportional to the square of the distance of the polarizable group and the double bond. Thus the allylic bond is expected to have a dominant effect because it is close to the double bond, and also because other groups may be sufficiently flexible for their effects to be canceled by virtue of random orientation. Among the three sets of anisotropies, two yielded the same signs as the Scott Wrixon rule42 and Anderson’s model56 as well. The authors admitted that too many assumptions were made to justify

4. The chiroptical properties of the carbon |
|
carbon double bond |
149 |
|
the above-mentioned rules and that the negative C C single-bond anisotropies which they have used represent only a minority viewpoint.
A second theoretical study, which was also based on the dynamic coupling mechanism, was developed by Weigang75. The formalism was developed for electric-dipole allowed transitions. Unlike most sector rules, Weigang’s formalism required75 careful observation of the orientation as well as the location of bonds placed dissymmetrically with respect to the chromophore. The expression representing the rotatory strength consists of two sector rules. In the first the space is divided into eight octants, while the second has a conical distribution which is depicted in Figure 10. In addition, the expression contained the averaged polarizability and the anisotropy of the perturber’s polarizability. This treatment was applied to the ethylenic chromophore, and (C)-trans-cyclooctene as well as twistene were examined in light of the two sector rules. The contributions to the sign of the ! Ł signal in (C)-trans-cyclooctene, from the conical and the octant sector rules, were both positive, which was the measured sign for this transition8. The predicted
FIGURE 10. Sectors and nodal surfaces for the two terms developed in Weigang’s theory. The signs refer to the absolute sign of the rotatory strength when the trigonometric functions and the ˛ˇ are all positive. The chromophore and perturber must be oriented in a manner discussed in Weigang’s paper and depicted in his Figure 175

150 |
Aharon Gedanken |
sign75 for the same transition in ( )-˛-pinene is positive, while a negative sign was measured8.
V. ISOTOPE EFFECT
Since the discovery of deuterium, many chiral compounds owing their optical activity to the presence of this isotope have been prepared76. In the early days of optical activity it was thought that such a small effect would not be amenable to experimental observation. Over the years it became clear that, for many chromophores, optical activity which is due solely to the introduction of a deuterium atom can be measured. In fact, the anisotropy factor, g, for the oxirane chromophore, for example, was a factor of 2 smaller when the deuterium was replaced by a methyl and almost the same as for a hydroxyl replacement77. The first report of CD measurements of olefins which owed their chirality solely to isotopic substitution was by Paquette and coworkers78. Four such compounds were prepared and measured in pentane solution at 20 °C. They were (1R)-[1-2H]-apobornene 11, (1R)-[1,2- 2H]-apobornene 13, (1R)-[2-2H]-apobornene 16 and (1R)-[2-2H]-norbornene 21. For two compounds, 13 and 21, gas-phase measurements were also performed and yielded the same results as in the pentane solution. The only exception was that the gas-phase absorption of compound 21 revealed78 two vibrational shoulders at 48600 and 49900 cm 1. The frequency interval between the two shoulders and their position on the red side of the main absorption band indicated that they arise from the ! 3s Rydberg transition. This Rydberg is overlaid by the ! Ł transition. The Rydberg has not been detected in the CD spectra of all the four compounds. The specific rotation of compounds 11 and 13 were negative, opposite to the CD sign of the lowest-energy band. The negative specific rotation was attributed to the higher excited states such as the ! yŁ , which indeed has a negative CD signal. The two isotopic perturbations in compound 13, which confer chirality upon the otherwise achiral apobornene structure, are different in kind. A comparison of the CD spectra of 11 and 22 (which has a methyl group in position 1) showed that the contributions of the C D and C CH3 in 1-position to the optical activity of the ! Ł are dissignate. This is similar to what was observed for carbonyls and other chromophores76. The comparison of two other compounds (21 and 23) which have a deuterium vs CH3 at the 2-position of norbornene showed consignate contributions to the
|
! |
Ł transition. The chiral perturbations introduced by C-1 and by C-2 in the chiral |
|||
|
|
|
78 |
that for the dissignate |
|
parent structure are of a different kind. The explanation was |
|
||||
effect of C-1, in addition to the usual common explanations |
|
the polarizability and the |
|||
|
bond-length changes the steric compression factor in the H/D isotopic replacement is also of importance. In other words the lighter isotope effectively acts like a larger atom in repulsive interactions with its neighbors, so that the bridgehead C C C bond angles are less compressed sterically in the 1-deuterio derivatives.
D
D |
D |
D |
|
D |
|
|
|
||
(11) |
|
(13) |
(16) |
(21) |
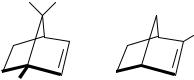
4. The chiroptical properties of the carbon |
|
carbon double bond |
151 |
|
CH3
CH3
(22) |
(23) |
For the 2-position in which the deuterium showed a consignate signal with the CH3 group, the explanation was centered on the displacement of the carbon atom of the olefin chromophore. Although the amplitude of the vibrational motion of a C H bond is larger than that of the corresponding C D bond, the displacement of the carbon atom in the motion is smaller for the lighter isotope. The mass of the methyl group is still larger, and the consignate effect of the C D and the C CH3 is thus dependent on the displacement of the carbon atom, which was related to the mass of the substituent.
We have already discussed another example63 of an isotope effect related to the CDC chromophore and the theoretical explanation72 which was proposed for the experimental results. It is worth mentioning that the ε/ε for the ! Ł transition of the deuteriosubstituted 4-methylcyclohexylidene was 4.4 ð 10 4, whereas for a methyl replacing a vinylic hydrogen a value of 1.6 ð 10 3 was obtained.
VI. LINEAR DICHROISM MEASUREMENTS
The spectroscopic properties of isolated double bonds were measured through their linear dichroism. The molecules were oriented in stretched films, and their UV spectra were measured using polarized light in the direction of stretching and orthogonal to it79. The instrumentation and the method by which these experiments were carried out was described in an early paper80. The molecules subjected to these measurements included symmetrically substituted and unsymmetrically substituted olefins. The symmetric molecule was bicyclohexylidene and among the optically active olefins were 4- and 5-cholestenes. While the first showed two bands at 208 and 185 nm, the latter revealed only one band peaked at 190 nm. The latter exhibited a wavelength-dependent linear dichroic spectrum, while bicyclohexylidene’s spectrum was wavelength-independent over the absorption bands. The two transitions were assigned as being polarized along the double bond. The broad and structureless band was resolved into two overlapping bands, 1 and 2, at shorter and longer wavelengths, respectively. The polarization of the shorter-wavelength component,1, was along the double-bond axis. The 2 transition formed an angle of 17° with the plane of the double bond; the projection of this transition on the double-bond plane falls at an angle of 10° with the axis of the double bond. The shorter-wavelength band, 1, was assigned as the valence ! Ł transition. The other band was assigned as the Rydberg! 3py , which is also known as the ! yŁ . The reader is, however, reminded that
the order of these excited states in Mason and Schnepps’ paper was reversed8.
VII. REFERENCES
1.E. Bunnenberg, C. Djerassi, K. Mislow and A. Moskowitz, J. Am. Chem. Soc., 84, 2823 (1962).
2.S. F. Mason and G. W. Vane, Chem. Commun., 540 (1965).
3.O. Schnepp, S. Allen and E. F. Pearson, Rev. Sci. Instrum., 41, 1136 (1970).
4.W. C. Johnson, Jr., Rev. Sci. Instrum., 42, 1283 (1971).

152 |
Aharon Gedanken |
5.A. J. Merer and R. S. Mulliken, Chem. Rev., 69, 639 (1969).
6.A. I. Scott and A. D. Wrixon, Chem. Commun., 1182 (1969).
7.A. Rauk and J. M. Barriel, Chem. Phys., 25, 409 (1977).
8.M. G. Mason and O. Schnepp J. Chem. Phys., 59, 1092 (1973).
9.M. Yaris, A. Moskowitz and R. S. Berry, J. Chem. Phys., 49, 3150 (1968).
10.A. I. Scott and A. D. Wrixon, Chem. Commun., 43 (1970).
11.R. D. Bach, J. Chem. Phys., 52, 6423 (1970).
12.O. Schnepp, E. F. Pearson and E. Sherman, J. Chem. Phys., 52, 6424 (1970).
13.M. B. Robin, H. Basch, N. A. Kuebler, B. E. Kaplan and J. Meinwald, J. Chem. Phys., 48, 5036 (1968).
14.C. C. Levin and R. Hoffmann, J. Am. Chem. Soc., 94, 3446 (1972).
15.E. Charney, H. Ziffer and U. Weiss, Tetrahedron, 21, 3121 (1965).
16.U. Weiss, H. Ziffer and E. Charney, Tetrahedron, 21, 3105 (1965).
17.M. G. Mason and O. Schnepp, J. Chem. Phys., 59, 1092 (1973).
18.R. J. Buenker, S. D. Peyerimhoff and W. E. Kammer, J. Chem. Phys., 55, 814 (1971).
19.T. D. Bouman and A. E. Hansen, J. Chem. Phys., 66, 3460 (1977).
20.A. Rauk, J. O. Jarvie, H. Schimura and J. M. Barriel, J. Am. Chem. Soc., 97, 5656 (1975).
21.D. H. Liskow and G. A. Segal, J. Am. Chem. Soc., 100, 2945 (1978).
22.A. E. Hansen and T. D. Bouman, Mol. Phys., 37, 1713 (1979).
23.A. E. Hansen and T. D. Bouman, J. Am. Chem. Soc., 107, 4828 (1985).
24.K. L. Bak, A. E. Hansen, K. Ruud, T. Helgaker, J. Olsen and P. Jorgensen, Theor. Chim. Acta, 90, 441 (1995).
25.M. Traetteberg, Acta Chem. Scand., B29, 29 (1975).
26.J. G. Kirkwood, J. Chem. Phys., 5, 479 (1937).
27.J. G. Kirkwood, J. Chem. Phys., 7, 139 (1939).
28.R. Rossi and P. Diversi, Tetrahedron, 26, 5033 (1970).
29.M. Levi, D. Cohen, V. Schurig, H. Basch and A. Gedanken, J. Am. Chem. Soc., 102, 6972 (1980).
30.C. F. Chabalowsky, G. M. Maggiora and R. E. Christoffersen, J. Am. Chem. Soc., 107, 1632 (1985).
31.K. P. Gross and O. Schnepp, Chem. Phys. Lett., 36, 531 (1975)
32.M. B. Robin, Higher Excited States of Polyatomic Molecules, Vol. I, Academic Press, New York, 1975, pp. 18 31 and 76 91.
33.A. F. Drake and S. F. Mason, Chem. Commun., 253 (1973).
34.I. Messing, B. Raz and J. Jortner, Chem. Phys., 25, 55 (1977).
35.G. Ya. Zelikina and T. G. Meister, Opt. Spectra, 42, 621 (1977).
36.G. Ya. Zelikina and T. G. Meister, Opt. Spectra, 43, 46 (1977).
37.A. F. Drake and S. F. Mason, Tetrahedron, 33, 937 (1977).
38.P. A. Snider and L. B. Clark, J. Chem. Phys., 52, 998 (1970).
39.W. Kuhn, Trans. Faraday Soc., 26, 293 (1930).
40.B. A. Arbuzv and V. A. Naumov, Dokl. Akad. Nauk SSSR, 158, 376 (1964).
41.P. Brint, E. Meshulam and A. Gedanken, Chem. Phys. Lett., 109, 383 (1984).
42.A. I. Scott and A. D. Wrixon, Tetrahedron, 26, 3695 (1970).
43.J. A. Schellman, J. Chem. Phys., 44, 55 (1966).
44.C. Djerassi, W. Klyne, T. Norin, G. Ohloff and E. Klein, Tetrahedron, 21, 163 (1965).
45.A. I. Vogel, J. Chem. Soc., 1833 (1948).
46.A. I. Vogel, W. J. Creswell, G. H. Jeffery and J. Leicester, J. Chem. Soc., 514 (1952).
47.M. Fetizon and I. Hanna, Chem. Commun., 462 (1970).
48.J. K. Gawronski and M. A. Kielczewski, Tetrahedron Lett., 2493 (1971).
49.A. Jogev, J. Sagiv and Y. Mazur, Chem. Commun., 411 (1972).
50.D. N. Kirk and R. J. Mullins, J. Chem. Soc., Perkin Trans, 1, 14 (1974).
51.M. Fetizon, I. Hanna, A. I. Scott, A. D. Wrixon and T. K. Devon, Chem. Commun., 545 (1971).
52.A. Yogev, D. Amar and Y. Mazur, Chem. Commun., 339 (1967).
53.A. W. Burgstahler and R. C. Barkhurst, J. Am. Chem. Soc., 92, 7601 (1970).
54.J. K. Gawronski and M. A. Kielczwski, Tetrahedron Lett., 2493 (1971).
55.W. Hug and G. Wagniere, Tetrahedron, 28, 1241 (1972).
56.N. H. Anderson, C. R. Costin, D. D. Syrdal D. P. Svedberg, J. Am. Chem. Soc., 95, 2049 (1973).
57.N. H. Anderson, C. R. Costin and J. R. Shaw, J. Am. Chem. Soc., 96, 3692 (1974).
58.M. Tichy, Tetrehedron Lett., 2001 (1972).
4. The chiroptical properties of the carbon |
|
carbon double bond |
153 |
|
59.J. Hudec and D. N. Kirk, Tetrahedron, 32, 2475 (1976).
60.A. I. Scott and A. D. Wrixon, Tetrahedron, 27, 4787 (1971).
61.J. A. Mills, J. Chem. Soc., 4976 (1952).
62.J. H. Brewster, J. Am. Chem. Soc., 81, 5493 (1959).
63.A. Gedanken, M. Duraisamy, J. Huang, J. Rachon and H. M. Walborsky, J. Am. Chem. Soc., 110, 4593 (1988).
64.M. B. Robin, Higher Excited States of Polyatomic Molecules, Vol. II, Academic Press, New York, 1975, pp. 26, 27 and 30.
65.D. A. Demo, Thesis, UCLA, 1969.
66.W. L. Meyerand and A. P. Loba, J. Am. Chem. Soc., 83, 4609 (1961).
67.D. A. Lightner and J. A. Gawronski and T. D. Bouman, J. Am. Chem. Soc., 102, 1983 (1980).
68.C. S. Barnes and C. Djerassi, J. Am. Chem. Soc., 84, 1962 (1962).
69.T. D. Bouman and A. J. Moskowitz, J. Chem. Phys., 48, 3115 (1968).
70.S. F. Mason, Molecular Optical Activity and Chiral Discrimination, Cambridge University Press, Cambridge, 1982.
71.E. Charney, The Molecular Basis of Optical Activity, Chap. 4, Wiley, New York, 1979.
72.A. Rodger, A. Gedanken and H. Klein, Mol. Phys., 72, 803 (1991).
73.A. I. Scott and C. Y. Yeh, J. Chem. Soc., Faraday Trans. 2, 71, 447 (1975).
74.I. Tinoco, Adv. Chem. Phys., 4, 113 (1962).
75.O. E. Weigang, J. Am. Chem. Soc., 101, 1965 (1979).
76.G. Barth and C. Djerassi, Tetrahedron, 37, 4123 (1981).
77.S. Ben-Tzur, A. Basil, A. Gedanken, J. A. Moore and J. M. Schwab, J. Am. Chem. Soc., 114, 5751 (1992).
78.L. A. Paquette, C. W. Doecke, F. R. Kearney, A. F. Drake and S. F. Mason, J. Am. Chem. Soc., 102, 7228 (1980).
79.A. Yogev, J. Sagiv and Y. Mazur, J. Am. Chem. Soc., 94, 5122 (1972).
80.(a) A. Yogev, L. Margulis, D. Amar and Y. Mazur, J. Am. Chem. Soc., 91, 4558 (1969).
(b)A. Yogev, J. Riboid, J. Marero and Y. Mazur, J. Am. Chem. Soc., 91, 4559 (1969).
(c)A. Yogev, L. Margulis and Y. Mazur, J. Am. Chem. Soc., 92, 6059 (1970).
(d)A. Yogev, L. Margulis and Y. Mazur, Chem. Phys. Lett., 8, 157 (1971).