
Physics of biomolecules and cells
.pdf
E. Sackmann et al.: Physics of Composite Cell Membranes |
279 |
actively pulled back provided the applied pressure is kept below a second threshold pressure pth2.
Initially only the lipid/protein bilayer together with a thin layer of actin is pulled into the pipette while the intracellular compartments are hold back by the bulk of the actin shell (cf. Fig. 21b). This fraction of the actin cortex forms a closed shell. It holds back the intracellular compartments which are aspired only at a later time if the suction pressure is high.
Dictyostelia cells exhibit several advantages for micromechanical studies of the cell cytoskeleton and the composite shell (cf. Schleicher & Noegel 1992). The cytoskeleton can be modified in a controlled way by removing one or several of the actin-binding proteins by mutations. Moreover, distinct cytoskeletal proteins can be fluorescent labelled by fusion with green fluorescent proteins through transfection. The shape of the cells can be controlled by starving the cells for distinct periods of time (cf. Schleicher & Noegel 1992). Moving cells exhibit transient polarities and the momentary front and rear of the cells may be distinguished as follows: the locomotion of amoeba like (rounded) Dictyostelia cells is associated with three distinct shape changes (cf. Schindl et al. 1995). First a thin lobe of the cell spreads over the surface for about 10 s forming a flat protrusion (pseudopod) of about 5 µm length. After formation of a new actin cortex in the protruded lobe the trailing end retracts (at fixed position of the leading edge of the pseudopod) and the cell assumes a buldged shape resulting in a strong reduction of the contact area. For several tens of seconds the cell persists in this resting state (typically for 100 s) before a new pseudopod forms in a new direction. In this way the leading front and the trailing end may be distinguished to study the micromechanical properties at sites of distinct activity within the cells. The micropipette experiments show (cf. Simson et al. 2001)
•The threshold pressure pth1 and thus the coupling strength of the membrane to the actin cortex is remarkably smaller (by 50%) at the
leading edge than at the trailing end. The length lc for a given pressure is about twice as large at the leading front;
•The maximum protrusion length increases roughly linearly with the applied suction pressure;
•The velocity of the advancing protrusion increases linearly with the suction pressure enabling estimates of the viscosity of the composite cell envelope;
•The aspired cell lobe is retraced again after a recovery time of 20 s, however only against suction pressures smaller than pth2 ≈ 1000 Pa;
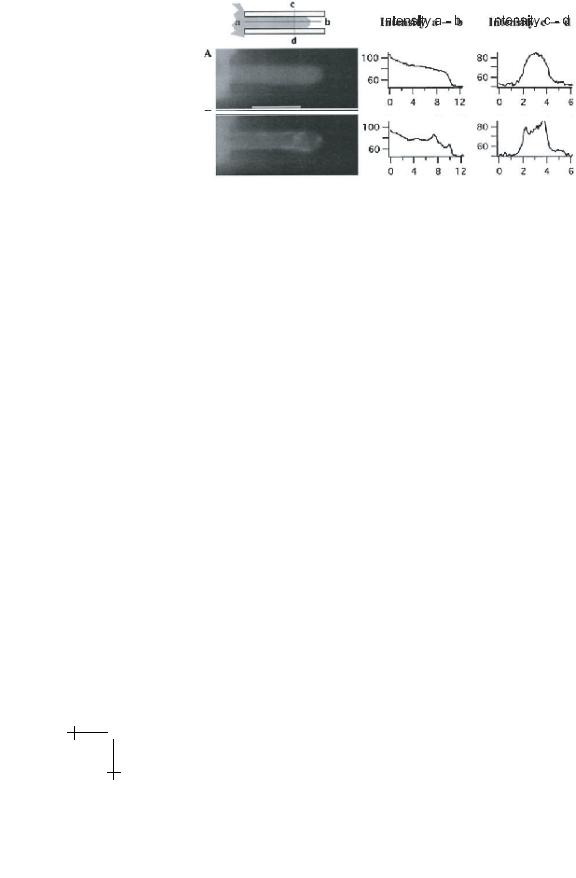

E. Sackmann et al.: Physics of Composite Cell Membranes |
281 |
myosin II increases the threshold pressure required for the aspiration of the protrusions by a factor of five that is from pth = 210 Pa for wild type cells to pth = 1000 Pa for the mutant. In agreement with the results obtained with magnetic bead microrheometry (cf. Sect. 6) this observation shows that the motor protein acts as softener of the actin-based cytoskeleton.
Conclusion: the envelopes of eucaryontic cells are soft, trilamellar shells composed of the central lipid protein bilayer (the biomembrane), the glycocalix, covering the outer surface and the cortex associated with the inner leaflet of the membrane. This composite membrane mediates local structural changes of the glycocalix (for the membrane) and the membraneassociated macromolecular cortex, which plays an essential role for the signal transduction between extracellular space during numerous cellular processes. In this lecture we discussed the physical properties of lipid membranes, the viscoelastic properties of the ultrasoft quasi-twodimensional networks of red blood cells and of pure cytoskeletal networks such as the semiflexible polymer actin and the regulation of the viscoelastic properties of composite membranes of eucaryontic cells.
The red blood cell exhibits outstanding elastic properties unmatched by technical materials: it is extremely soft with respect to bending and shearing but hard as solids towards extensions which is essential for the survival of the cells during their several hundred kilometer long journey through the capillary system. These unique properties are due to the ultrasoft quasitwodimensional triangular network (mesh size 80 nm) with the sides formed by flexible spectrin filaments and corners formed by actin oligomers.
Pure Actin networks are also of great interest from the point of view of polymer physics. Since the contour lengths (20 m) and persistence lengths (10 m) are easily accessible to the optical microscopy one can visualize and analyze the conformational dynamics, the local and long range di usivity and the flexibility of single filaments by microflueorescence or the analysis of the Brownian motion of filament coupled colloidal gold. Together with novel colloidal bead microrheometry techniques such studies open the possibility to relate molecular motional properties of the local structural fluctuations to the macroscopic viscoelastic impedance.
We discussed the self-assembly and function of the endothelial cell monolayers lining the inner wall of blood vessels which implies two fundamental and vital processes, cell adhesion (treated in the accompanying article by Sackmann and Bruinsma) and numerous chemo-mechanical processes. The manifold functions of the actin based cytoskeleton (e.g. during numerous chemomechanical processes) is determined by the outstanding viscoelastic properties of this prototype of a semiflexible macromolecule. A Phallanx of actin manipulating proteins (including motor proteins of the myosin family) together with the regulation of their activity by second messengers enables

282 |
Physics of Bio-Molecules and Cells |
cells to switch rapidly between di erent local structural organizations of the cytoskeleton. Heterogeneous gels can be rapidly formed or reorganized (in sub-second time scales) through phase transitions such as the formation of heterogels composed of densely packed domains (microgels) interconnected by single filaments or bundles from homogeneous networks by switching on the activity of cross-linkers (e.g. a-actinin). Such percolated networks combine astonishingly high stifnesses with lage soft voids enabling the uptake and e ective transpot of particles. They can transform further into states of bundle-network coexistence.
Magnetic bead microrheometry studies demonstrate that the cytoplasm exhibits typical viscoelastic features of such heterogeneous networks with two fundamental di erences. The heteogels are highly dynamic and thus behave as soft viscoelastic bodies and the active transport of intracellular phagosomes is thus determined by a force dependent mobility. The cells can respond to extrinsic forces by adaptive active reorganizations of the cytoskeleton thus facilitating the intracellular transport.
These examples demonstrate the continuous need for new physical techniques, which allow the local manipulation of biomaterials to understand their unique physical properties. The study of their complexity and their heterongenety are not only of fundamental physical interest but also allow the quantification and thus fundamental understanding of important biological processes.
References
[1]F. Amblard, A.C. Maggs, B. Yurke, A.N. Pargellis and S. Yeibler, Phys. Rev. Lett. 77 (1996) 4470.
[2]G. Bassell and R.H. Singer, Curr. Biol. 9 (1997) 109.
[3]A. Bausch, W. M¨oller and E. Sackmann, Biophys. J. 76 (1999) 573.
[4]T.M. Bayerl and E. Sackmann, Neutron scattering as a tool to probe the e ect of cholesterol on phase separation, structure formation and lipid protein interaction in model membranes. Cholesterol in Membranes, edited by L. Finegold (CRC Press, Boca Raton, USA, 1992).
[5]V. Bennet, Phys. Rev. 70 (1990).
[6]A. Bremer, R.C. Millonig, R. S¨utterlin, A. Engel, T.D. Polard and U. Aebi, J. Cell Biol. 115 (1991) 689.
[7]A. Bretscher, Curr. Op. Cell Biol. 11 (1999) 109.
[8]F. Brochard and J.F. Lennon, J. Phys. 11 (1975) 1035.
[9]P. de Gennes, Scaling concepts in polymer physics (Cornell University Press, Ithaca, 1979).
[10]M. Dichtl and E. Sackmann, New J. Phys. 1 (1999) 181.
[11]H.G. D¨obereiner, J. K¨as, D. Noppl, I. Sprenger and E. Sackmann, Biophys. J. 65 (1993) 1396.
[12]M. Doi and S.F. Edwards, The theory of polymer dynamics (Clarendon Press, Oxford, 1986).

E. Sackmann et al.: Physics of Composite Cell Membranes |
283 |
[13]H.P. Duwe and E. Sackmann, Physica 163 (1990) 410.
[14]H. Engelhardt and E. Sackmann, Biophys. J. 54 (1988) 267.
[15]J.C. Crocker et al., Phys. Rev. Lett. 85 (2000) 888.
[16]E.A. Evans, Biophys. J. 14 (1972) 923.
[17]E.A. Evans and D. Needham, Faraday Discussion Chem. Soc. 81 (1986) 267.
[18]E.A. Evans and W. Rawicz, Phys. Rev. Lett. 64 (1990) 2094.
[19]E.A. Evans and A. Yeung, Biophys. J. 56 (1989) 151.
[20]W. Feneberg, M. Westphal and E. Sackmann, Eur. Biophys. J. 30 (2001) 284.
[21]J.D. Ferry, Viscoelastic properties of polymers (Wiley, London, 1980).
[22]E. Frey and J. Wilhelm, Phys. Rev. Lett. 77 (1996) 2581.
[23]J.G.N. Garcia and K.L. Schaphorst, J. Inv. Med. 43 (1995) 117.
[24]F. Gittes and F. MacKintosh, Phys. Rev. E 58 (1998) 1241.
[25]R. G¨otter, K. Kroy, E. Frey, M. B¨armann and E. Sackmann, Macromol. 29 (1996) 30.
[26]W. H¨ackl, U. Seifert and E. Sackmann, J. Phys. II 7 (1997) 1141.
[27]W. Helfrich and R.M. Servuss, Nuovo Cimento D 3D (1984) 137.
[28]H. Hillebrandt, A. Abdelghani, C. Abdelghani-Jacquin, M. Aepfelbacher and
E.Sackmann, Appl. Phys. A 73 (2001) 539.
[29]B. Hinner, M. Tempel, E. Sackmann, K. Kroy and E. Frey, Phys. Rev. Lett. 12 (1998) 2614.
[30]H. Isambert and A. C. Maggs, Macromol. 29 (1996) 1036.
[31]G. Isenberg, J. Mus. Res. Cell Mot. 12 (1991) 136.
[32]P. Janmey, Cell membranes and the cytoskeleton, volume 1B of Handbook of biological physics, edited by R. Lipowsky and E. Sackmann (Elsevier, Amsterdam, 1995), Chapter 11.
[33]A. Jungbluth, V. von Arnim, E. Biegelmann, B. Humbel, A. Schweiger and G. Gerish, J. Cell Sc. 107 (1994) 117.
[34]J. K¨as, H. Strey, J.X. Tang, D. Finger, R. Ezzel, E. Sackmann and P. Janmey,
Biophys. J. 70 (1996) 609.
[35]M. Keller, J. Schilling, and E. Sackmann, J. Sci. Instrum. 72 (2001) 3626.
[36]E. Korn, M. Cartier and D. Pantaloni, Science 238 (1987) 638.
[37]K. Kroy and E. Frey, Phys. Rev. Lett. 77 (1996) 306.
[38]L. Landau and E. Lifshitz, Theory of elasticity (Pergamon Press, 2nd edition, 1970).
[39]R. Lipowsky, Generic interactions of flexible membranes, volume 1B of Handbook of biological physics, edited by R. Lipowsky and E. Sackmann (Elsevier, Amsterdam, 1995), Chapter 11.
[40]R. Lipowsky and M. Girardet, Phys. Rev. Lett. 65 (1990) 2893.
[41]H. Lodish, Molecular cell biology (W.H. Freeman Company, New York, 1995).
[42]L. Machesky and K. Gould, Curr. Op. Cell Biol. 11 (1999) 117.
[43]F.C. MacKintosh, J. K¨as and P.A. Janmey, Phys. Rev. Lett. 75 (1995) 4425.
[44]A. McGough, B. Pope, W. Chiu and H. Weeds, J. Cell Biol. 138 (1997) 771.
[45]R. Merkel, R. Simson, D.A. Simson, M. Hohenadl, A. Boulbitch, E. Wallra and
E.Sackmann, Biophys. J. 79 (2000) 707.
[46]S.T. Millner and S.A. Safran, Phys. Rev. A 36 (1987) 4371.
[47]D. Morse, Macromol. 31 (1998) 7030.
[48]D. Morse, Phys. Rev. E 63 (2001).
[49]O. M¨uller, H.E. Gaub, M. B¨armann and E. Sackmann, Macromol. 24 (1991) 3111.

284 |
Physics of Bio-Molecules and Cells |
[50]T. Odijk, Macromol. 16 (1983) 1340.
[51]G. Oster, Cell Motil. Cytoskel. 10 (1988) 164.
[52]A. Palmer, T.G. Mason, J. Xu, S.C. Kuo and D. Wirtz, Biophys. J. 76 (1999) 1063.
[53]C. Pasternak, P. Flicker, S. Ravid and J.A. Spudich, J. Cell Biol. 109 (1998) 203.
[54]T. Piekenbrock and E. Sackmann, Biopolymers 32 (1992) 1471.
[55]J.L. Podolski and T. Steck, J. Biol. Chem. 103 (1986) 2747.
[56]A. Radhakrishnan and H.M. McConnell, Biophys. J. 77 (1999) 1507.
[57]J. R¨adler, T. Feder, H. Strey and E. Sackmann, Phys. Rev. E 51 (1995) 4526.
[58]E. Sackmann, FEBS Lett. 346 (1994) 3.
[59]E. Sackmann, Biological membranes: Architecture and Functions, volume 1A of
Handbook of biological physics, edited by R. Lipowsky and E. Sackmann (Elsevier, Amsterdam, 1995), Chapter 1.
[60]M. Schindl, E. Wallra , B. Deubzer, W. Witke, G. Gerish and E. Sacmann, Biophys.
J.68 (1995) 1177.
[61]M. Schleicher and A. Noegel, The New Biologist 4 (1992) 461.
[62]U. Seifert, Phys. Rev. Lett. 74 (1995) 5060.
[63]T.A. Springer, Cell 76 (1994) 301.
[64]H. Strey, M. Peterson and E. Sackmann, Biophys. J. 69, (1995) 478.
[65]M. Tempel, W.H. Goldmann, C. Dietrich, V. Niggli, T. Weber, E. Sackmann and
G.Isenberg, Biochemistry 33 (1994) 12565.
[66]M. Tempel, G. Isenberg and E. Sackmann, Phys. Rev. E 54 (1996) 1802.
[67]S. Tuvia, A. Moses, N. Gulayev, S. Levin and R. Korenstein, J. Phys. 516 (1999) 781.
[68]J. Wegener, S. Zink, D. R¨osen and H.J. Galla, Eur. J. Physiol. 437 (1999) 925.
[69]D.V. Zhelev and R. Hochmut, Biophys. J. 68 (1995) 2004.
[70]F. Ziemann, J. R¨adler and E. Sackmann, Biophys. J. 66 (1994) 2210.
[71]A. Zilker, M. Ziegler and E. Sackmann, Phys. Rev. A 46 (1992) 7998.
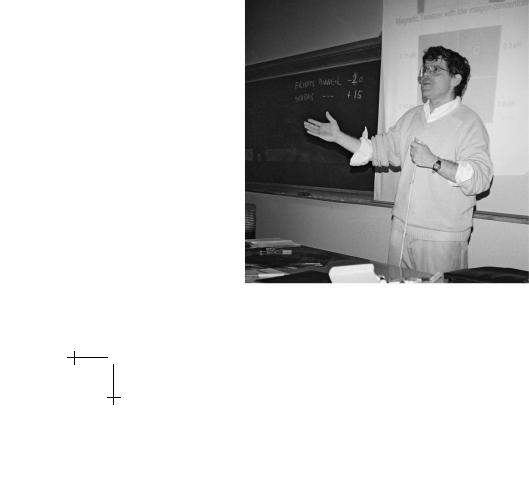
COURSE 7
CELL ADHESION AS WETTING TRANSITION?
E. SACKMANN AND R. BRUINSMA
Physik Department E22,
James-Franck-Straße, D-85748 Garching b. M¨unchen, GermanyUniversiteit Leiden, Instituut-Lorentz voor Theoretische Natuurkunde, Postbus 9506, NL-2300 RA Leiden, The Netherlands

Contents
1 |
Introduction |
287 |
2 |
Mimicking cell adhesion |
292 |
3 |
Microinterferometry: A versatile tool to evaluate adhesion strength |
|
|
and forces |
294 |
4 |
Soft shell adhesion is controlled by a double well interfacial potential |
294 |
5 |
How is adhesion controlled by membrane elasticity? |
297 |
6 |
Measurement of adhesion strength by interferometric contour |
|
|
analysis |
299 |
7 |
Switching on specific forces: Adhesion as localized dewetting process |
300 |
8 |
Measurement of unbinding forces, receptor-ligand leverage |
|
|
and a new role for stress fibers |
300 |
9 |
An application: Modification of cellular adhesion strength |
|
|
by cytoskeletal mutations |
303 |
10 |
Conclusions |
303 |
A |
Appendix: Generic interfacial forces |
304 |
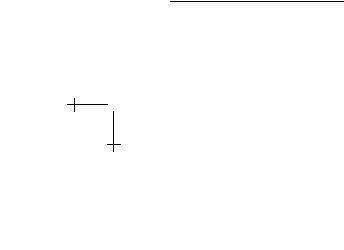
CELL ADHESION AS WETTING TRANSITION?
E. Sackmann1 and R. Bruinsma2
Abstract
Cell adhesion is controlled by a complex interplay of short range (lock- and-key) forces mediated by cell surface receptors, a phalanx of (short and long range) non-specific (generic) interactions and, last but not least, membrane elasticity. The physical basis of cell adhesion is explored by the design of simplified model systems mimicking cell and tissue surfaces enabling local measurements of cellular shape changes and adhesion forces by microinterferometry. Cell adhesion can be understood as first order dewetting transition resulting in the formation of adhesion plaques such as focal adhesion sites of cells which allows cell adhesion at astonishingly low receptor densities. The repeller molecules of the glycocalix play a key role for the control of the adhesion transition and the mechanical stability of the adhering cells by relaxing the strength of the binding forces. Stress fibers are postulated to be essential for the stabilization of adhesion domains against leverage through bending moments enforced by hydrodynamic shear forces.
1 Introduction
Cell adhesion is a fascinating albeit very complex biological process which controls many life processes. Examples are the formation of specific organs during the magic process of the transformation of fertilized eggs into embryos or the assembly of endothelial cells into tubular structures during the formation of blood vessels or of the blood-brain barrier [1]. The specificity of cell adhesion is controlled by genetic expression of receptors at the cell surface which bind specifically to adhesion molecules of target cells or tissue by the lock-and-key force principle. However, as illustrated in Figure 1, cell adhesion is also controlled by a manifold of generic forces and, most importantly, by membrane elasticity. The situation is further obscured by often
1Physik Department E22, James-Franck-Straße, D-85748 Garching b. M¨unchen, Germany.
2Universiteit Leiden, Instituut-Lorentz voor Theoretische Natuurkunde, Postbus 9506, NL-2300 RA Leiden, The Netherlands.
c EDP Sciences, Springer-Verlag 2002
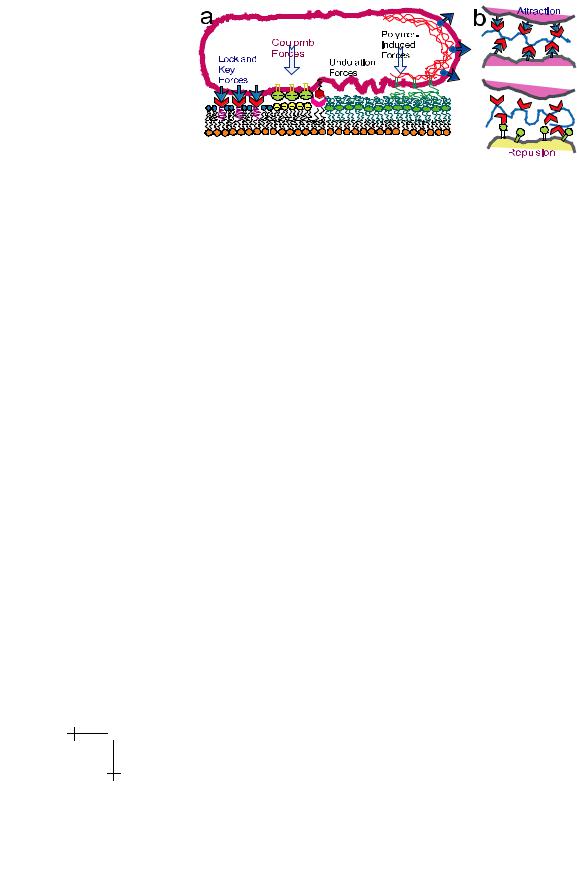
288 |
|
|
|
|
|
Physics of Bio-Molecules and Cells |
|
|
|
|
|
|
|
|
||||||||||||
|
|
|
|
|
|
|
|
|
|
|
|
|
|
|
|
|
|
|
|
|
|
|
|
|
|
|
|
|
|
|
|
|
|
|
|
|
|
|
|
|
|
|
|
|
|
|
|
|
|
|
|
|
|
|
|
|
|
|
|
|
|
|
|
|
|
|
|
|
|
|
|
|
|
|
|
|
|
|
|
|
|
|
|
|
|
|
|
|
|
|
|
|
|
|
|
|
|
|
|
|
|
|
|
|
|
|
|
|
|
|
|
|
|
|
|
|
|
|
|
|
|
|
|
|
|
|
|
|
|
|
|
|
|
|
|
|
|
|
|
|
|
|
|
|
|
|
|
|
|
|
|
|
|
|
|
|
|
|
|
|
|
|
|
|
|
|
|
|
|
|
|
|
|
|
|
|
|
|
|
|
|
|
|
|
|
|
|
|
|
|
|
|
|
|
|
|
|
|
|
|
|
|
|
|
|
|
|
|
|
|
|
|
|
|
|
|
|
|
|
|
|
|
|
|
|
|
|
|
|
|
|
|
|
|
|
|
|
|
|
|
|
|
|
|
|
|
|
|
|
|
|
|
|
|
|
|
|
|
|
|
|
|
|
|
|
|
|
|
|
|
|
|
|
|
|
|
|
|
|
|
|
|
|
|
|
|
|
|
|
|
|
|
|
|
|
|
|
|
|
|
|
|
|
|
|
|
|
|
|
|
|
|
|
|
|
|
|
|
|
|
|
|
|
|
|
|
|
|
|
|
|
|
|
|
|
|
|
|
|
|
|
|
|
|
|
|
|
|
|
|
|
|
|
|
|
|
|
|
|
|
|
|
|
|
|
|
|
|
|
|
|
|
|
|
|
|
|
Fig. 1. Control of cell adhesion by interplay of receptor-mediated specific forces, a manifold of generic interfacial forces and membrane elasticity. The cartoon shows the interaction of a cell with a biofunctional surface mimicking target cells or tissue. Non specific forces include: (i) electrostatic interactions which can be mediated by cell surface receptors exhibiting large numbers of acidic sialic acid residues (cf. GlyCAM, Fig. 3); (ii) attractive Van der Waals interactions, (iii) repulsive undulation forces due to thermally excited bending undulations (flickering) of lipid protein bilayers; (iv) a manifold of polymer induced forces. The latter may include steric repulsion forces between molecules of the glycocalix such as cell adhesion molecules exhibiting long extracellular chains (cf. Fig. 3) but also interactions mediated by extracellular matrix proteins such as hyaluronic acid and fibronectin which are bound to their respective receptors CD44 and integrin, respectively. These interactions can be attractive if the opposing cells (or cells and tissue-surfaces) exhibit receptors for the macromolecules but will be repulsive in the absence of such receptors. Note that fibronectin has also a binding domain for collagen IV a major component of the basal membrane forming the top layer of endothelial cells.
drastic reorganizations of the membrane-coupled, actin-based cytoskeleton induced by receptor-ligand recognition. A prominent example is the formation of focal adhesion complexes formed by a phalanx of actin binding proteins mediating the formation of actin bundles (stress fibers) and their coupling to the cytoplasmic domains of membrane spanning cell surface receptors [1].
Studies of adhesion processes are further hampered by the fact that the formation of stable cell-cell and cell-tissue contacts is a dynamic process involving a sequence of steps [2].
Firstly, as shown in Figure 2, the receptors and ligands are often hidden within the several ten nm thick glycocalix. It is formed mainly by membrane anchored cell surface molecules with very large head groups such as cell adhesion molecules (CAMs) exhibiting head groups which may extend by up