
- •Foreword
- •Preface
- •Contents
- •Introduction
- •Oren M. Becker
- •Alexander D. MacKerell, Jr.
- •Masakatsu Watanabe*
- •III. SCOPE OF THE BOOK
- •IV. TOWARD A NEW ERA
- •REFERENCES
- •Atomistic Models and Force Fields
- •Alexander D. MacKerell, Jr.
- •II. POTENTIAL ENERGY FUNCTIONS
- •D. Alternatives to the Potential Energy Function
- •III. EMPIRICAL FORCE FIELDS
- •A. From Potential Energy Functions to Force Fields
- •B. Overview of Available Force Fields
- •C. Free Energy Force Fields
- •D. Applicability of Force Fields
- •IV. DEVELOPMENT OF EMPIRICAL FORCE FIELDS
- •B. Optimization Procedures Used in Empirical Force Fields
- •D. Use of Quantum Mechanical Results as Target Data
- •VI. CONCLUSION
- •REFERENCES
- •Dynamics Methods
- •Oren M. Becker
- •Masakatsu Watanabe*
- •II. TYPES OF MOTIONS
- •IV. NEWTONIAN MOLECULAR DYNAMICS
- •A. Newton’s Equation of Motion
- •C. Molecular Dynamics: Computational Algorithms
- •A. Assigning Initial Values
- •B. Selecting the Integration Time Step
- •C. Stability of Integration
- •VI. ANALYSIS OF DYNAMIC TRAJECTORIES
- •B. Averages and Fluctuations
- •C. Correlation Functions
- •D. Potential of Mean Force
- •VII. OTHER MD SIMULATION APPROACHES
- •A. Stochastic Dynamics
- •B. Brownian Dynamics
- •VIII. ADVANCED SIMULATION TECHNIQUES
- •A. Constrained Dynamics
- •C. Other Approaches and Future Direction
- •REFERENCES
- •Conformational Analysis
- •Oren M. Becker
- •II. CONFORMATION SAMPLING
- •A. High Temperature Molecular Dynamics
- •B. Monte Carlo Simulations
- •C. Genetic Algorithms
- •D. Other Search Methods
- •III. CONFORMATION OPTIMIZATION
- •A. Minimization
- •B. Simulated Annealing
- •IV. CONFORMATIONAL ANALYSIS
- •A. Similarity Measures
- •B. Cluster Analysis
- •C. Principal Component Analysis
- •REFERENCES
- •Thomas A. Darden
- •II. CONTINUUM BOUNDARY CONDITIONS
- •III. FINITE BOUNDARY CONDITIONS
- •IV. PERIODIC BOUNDARY CONDITIONS
- •REFERENCES
- •Internal Coordinate Simulation Method
- •Alexey K. Mazur
- •II. INTERNAL AND CARTESIAN COORDINATES
- •III. PRINCIPLES OF MODELING WITH INTERNAL COORDINATES
- •B. Energy Gradients
- •IV. INTERNAL COORDINATE MOLECULAR DYNAMICS
- •A. Main Problems and Historical Perspective
- •B. Dynamics of Molecular Trees
- •C. Simulation of Flexible Rings
- •A. Time Step Limitations
- •B. Standard Geometry Versus Unconstrained Simulations
- •VI. CONCLUDING REMARKS
- •REFERENCES
- •Implicit Solvent Models
- •II. BASIC FORMULATION OF IMPLICIT SOLVENT
- •A. The Potential of Mean Force
- •III. DECOMPOSITION OF THE FREE ENERGY
- •A. Nonpolar Free Energy Contribution
- •B. Electrostatic Free Energy Contribution
- •IV. CLASSICAL CONTINUUM ELECTROSTATICS
- •A. The Poisson Equation for Macroscopic Media
- •B. Electrostatic Forces and Analytic Gradients
- •C. Treatment of Ionic Strength
- •A. Statistical Mechanical Integral Equations
- •VI. SUMMARY
- •REFERENCES
- •Steven Hayward
- •II. NORMAL MODE ANALYSIS IN CARTESIAN COORDINATE SPACE
- •B. Normal Mode Analysis in Dihedral Angle Space
- •C. Approximate Methods
- •IV. NORMAL MODE REFINEMENT
- •C. Validity of the Concept of a Normal Mode Important Subspace
- •A. The Solvent Effect
- •B. Anharmonicity and Normal Mode Analysis
- •VI. CONCLUSIONS
- •ACKNOWLEDGMENT
- •REFERENCES
- •Free Energy Calculations
- •Thomas Simonson
- •II. GENERAL BACKGROUND
- •A. Thermodynamic Cycles for Solvation and Binding
- •B. Thermodynamic Perturbation Theory
- •D. Other Thermodynamic Functions
- •E. Free Energy Component Analysis
- •III. STANDARD BINDING FREE ENERGIES
- •IV. CONFORMATIONAL FREE ENERGIES
- •A. Conformational Restraints or Umbrella Sampling
- •B. Weighted Histogram Analysis Method
- •C. Conformational Constraints
- •A. Dielectric Reaction Field Approaches
- •B. Lattice Summation Methods
- •VI. IMPROVING SAMPLING
- •A. Multisubstate Approaches
- •B. Umbrella Sampling
- •C. Moving Along
- •VII. PERSPECTIVES
- •REFERENCES
- •John E. Straub
- •B. Phenomenological Rate Equations
- •II. TRANSITION STATE THEORY
- •A. Building the TST Rate Constant
- •B. Some Details
- •C. Computing the TST Rate Constant
- •III. CORRECTIONS TO TRANSITION STATE THEORY
- •A. Computing Using the Reactive Flux Method
- •B. How Dynamic Recrossings Lower the Rate Constant
- •IV. FINDING GOOD REACTION COORDINATES
- •A. Variational Methods for Computing Reaction Paths
- •B. Choice of a Differential Cost Function
- •C. Diffusional Paths
- •VI. HOW TO CONSTRUCT A REACTION PATH
- •A. The Use of Constraints and Restraints
- •B. Variationally Optimizing the Cost Function
- •VII. FOCAL METHODS FOR REFINING TRANSITION STATES
- •VIII. HEURISTIC METHODS
- •IX. SUMMARY
- •ACKNOWLEDGMENT
- •REFERENCES
- •Paul D. Lyne
- •Owen A. Walsh
- •II. BACKGROUND
- •III. APPLICATIONS
- •A. Triosephosphate Isomerase
- •B. Bovine Protein Tyrosine Phosphate
- •C. Citrate Synthase
- •IV. CONCLUSIONS
- •ACKNOWLEDGMENT
- •REFERENCES
- •Jeremy C. Smith
- •III. SCATTERING BY CRYSTALS
- •IV. NEUTRON SCATTERING
- •A. Coherent Inelastic Neutron Scattering
- •B. Incoherent Neutron Scattering
- •REFERENCES
- •Michael Nilges
- •II. EXPERIMENTAL DATA
- •A. Deriving Conformational Restraints from NMR Data
- •B. Distance Restraints
- •C. The Hybrid Energy Approach
- •III. MINIMIZATION PROCEDURES
- •A. Metric Matrix Distance Geometry
- •B. Molecular Dynamics Simulated Annealing
- •C. Folding Random Structures by Simulated Annealing
- •IV. AUTOMATED INTERPRETATION OF NOE SPECTRA
- •B. Automated Assignment of Ambiguities in the NOE Data
- •C. Iterative Explicit NOE Assignment
- •D. Symmetrical Oligomers
- •VI. INFLUENCE OF INTERNAL DYNAMICS ON THE
- •EXPERIMENTAL DATA
- •VII. STRUCTURE QUALITY AND ENERGY PARAMETERS
- •VIII. RECENT APPLICATIONS
- •REFERENCES
- •II. STEPS IN COMPARATIVE MODELING
- •C. Model Building
- •D. Loop Modeling
- •E. Side Chain Modeling
- •III. AB INITIO PROTEIN STRUCTURE MODELING METHODS
- •IV. ERRORS IN COMPARATIVE MODELS
- •VI. APPLICATIONS OF COMPARATIVE MODELING
- •VII. COMPARATIVE MODELING IN STRUCTURAL GENOMICS
- •VIII. CONCLUSION
- •ACKNOWLEDGMENTS
- •REFERENCES
- •Roland L. Dunbrack, Jr.
- •II. BAYESIAN STATISTICS
- •A. Bayesian Probability Theory
- •B. Bayesian Parameter Estimation
- •C. Frequentist Probability Theory
- •D. Bayesian Methods Are Superior to Frequentist Methods
- •F. Simulation via Markov Chain Monte Carlo Methods
- •III. APPLICATIONS IN MOLECULAR BIOLOGY
- •B. Bayesian Sequence Alignment
- •IV. APPLICATIONS IN STRUCTURAL BIOLOGY
- •A. Secondary Structure and Surface Accessibility
- •ACKNOWLEDGMENTS
- •REFERENCES
- •Computer Aided Drug Design
- •Alexander Tropsha and Weifan Zheng
- •IV. SUMMARY AND CONCLUSIONS
- •REFERENCES
- •Oren M. Becker
- •II. SIMPLE MODELS
- •III. LATTICE MODELS
- •B. Mapping Atomistic Energy Landscapes
- •C. Mapping Atomistic Free Energy Landscapes
- •VI. SUMMARY
- •REFERENCES
- •Toshiko Ichiye
- •II. ELECTRON TRANSFER PROPERTIES
- •B. Potential Energy Parameters
- •IV. REDOX POTENTIALS
- •A. Calculation of the Energy Change of the Redox Site
- •B. Calculation of the Energy Changes of the Protein
- •B. Calculation of Differences in the Energy Change of the Protein
- •VI. ELECTRON TRANSFER RATES
- •A. Theory
- •B. Application
- •REFERENCES
- •Fumio Hirata and Hirofumi Sato
- •Shigeki Kato
- •A. Continuum Model
- •B. Simulations
- •C. Reference Interaction Site Model
- •A. Molecular Polarization in Neat Water*
- •B. Autoionization of Water*
- •C. Solvatochromism*
- •F. Tautomerization in Formamide*
- •IV. SUMMARY AND PROSPECTS
- •ACKNOWLEDGMENTS
- •REFERENCES
- •Nucleic Acid Simulations
- •Alexander D. MacKerell, Jr.
- •Lennart Nilsson
- •D. DNA Phase Transitions
- •III. METHODOLOGICAL CONSIDERATIONS
- •A. Atomistic Models
- •B. Alternative Models
- •IV. PRACTICAL CONSIDERATIONS
- •A. Starting Structures
- •C. Production MD Simulation
- •D. Convergence of MD Simulations
- •WEB SITES OF INTEREST
- •REFERENCES
- •Membrane Simulations
- •Douglas J. Tobias
- •II. MOLECULAR DYNAMICS SIMULATIONS OF MEMBRANES
- •B. Force Fields
- •C. Ensembles
- •D. Time Scales
- •III. LIPID BILAYER STRUCTURE
- •A. Overall Bilayer Structure
- •C. Solvation of the Lipid Polar Groups
- •IV. MOLECULAR DYNAMICS IN MEMBRANES
- •A. Overview of Dynamic Processes in Membranes
- •B. Qualitative Picture on the 100 ps Time Scale
- •C. Incoherent Neutron Scattering Measurements of Lipid Dynamics
- •F. Hydrocarbon Chain Dynamics
- •ACKNOWLEDGMENTS
- •REFERENCES
- •Appendix: Useful Internet Resources
- •B. Molecular Modeling and Simulation Packages
- •Index
488 |
Tobias |
begin to change place only after several nanoseconds in fluid phase bilayers [52], it is likely that Brownian lateral motion of lipids will not be observed until well beyond 10 ns.
Analysis of neutron data in terms of models that include lipid center-of-mass diffusion in a cylinder has led to estimates of the amplitudes of the lateral and out-of-plane motion and their corresponding diffusion constants. It is important to keep in mind that these diffusion constants are not derived from a Brownian dynamics model and are therefore not comparable to diffusion constants computed from simulations via the Einstein relation. Our comparison in the previous section of the Lorentzian line widths from simulation and neutron data has provided a direct, model-independent assessment of the integrity of the time scales of the dynamic processes predicted by the simulation. We estimate the amplitudes within the cylindrical diffusion model, i.e., the length (twice the out-of-plane amplitude) L and the radius (in-plane amplitude) R of the cylinder, respectively, as follows:
L 2 ∆z 2 |
(10) |
R ( ∆x 2 ∆y 2)1/2 |
(11) |
where ∆x 2, ∆y 2, and ∆z 2 are the mean-square fluctuations in the x, y, and z directions,
|
˚ |
˚ |
respectively. Averaging over 100 ps, we find L 1.3 A and R 0.96 A. These may be |
||
˚ |
˚ |
|
compared to the values L 2.3 A and R 1.1 A obtained in the model III/IV fits to
neutron time-of-flight data taken on IN5 by Ko¨nig et al. [64] on a DPPC bilayer at 60°C and 23% water. The cylinder radius derived from the simulation is slightly smaller than that derived from the neutron data, and the cylinder length is substantially shorter. This in contrast to our expectation that the simulation values should, if anything, be larger because the simulation was carried out at full hydration. Moreover, the simulation predicts an appreciable anisotropy that it absent from the experimental data. There are two possible explanations for the discrepancies. The first is that simulation models the lipid center-of- mass motion poorly. The second, which is probably more correct, is that the combined diffusion in a cylinder-and-chain defect model used to determine the L and R values quoted here attributes too little motion to the chains and too much to the center of mass. We examine models for the motion of the lipid internal degrees of freedom in the next section.
F. Hydrocarbon Chain Dynamics
Two physically reasonable but quite different models have been used to describe the internal motions of lipid molecules observed by neutron scattering. In the first the protons are assumed to undergo diffusion in a sphere [63]. The radius of the sphere is allowed to be different for different protons. Although the results do not seem to be sensitive to the details of the variation in the sphere radii, it is necessary to have a range of sphere volumes, with the largest volume for methylene groups near the ends of the hydrocarbon chains in the middle of the bilayer and the smallest for the methylenes at the tops of the chains, closest to the bilayer surface. This is consistent with the behavior of the carbon–deuterium order parameters, SCD, measured by deuterium NMR:
SCD |
1 |
3 cos2θ 1 |
(12) |
|
|||
2 |
|
|
where θ is the angle between the CED bond vector and the bilayer normal [7]. We show the negative of the usual order parameters, which may be thought of as ‘‘disorder parameters,’’ averaged over both chains in all the molecules in our bilayer simulation, in
Membrane Simulations |
489 |
Figure 14 Measures of disorder in the acyl chains from an MD simulation of a fluid phase DPPC bilayer, (a) Order parameter profile of the CEH bonds; (b) root-mean-square fluctuation of the H atoms averaged over 100 ps.
Figure 14a. Although our values are not in quantitative agreement with the experimental values (see Ref. 50 for a discussion), they faithfully reproduce the well-known observation that the disorder increases from the tops of the chains toward the middle of the bilayer, with a more pronounced increase in the last few carbons of the chain. The SCD represent different types of disorder, including chain conformational defects and whole molecule ‘‘wobbling’’ [67].
To make contact with the diffusion-in-a-sphere model, we have defined the spherical radius as the root-mean-square fluctuation of the protons averaged over 100 ps. The varia-

490 Tobias
tion of the spherical radius as a function of position in the chains is plotted in Figure 14b.
˚
As expected, the radius increases monotonically from a minimum value of 1.8 A at the
˚
tops of the chains to a maximum of 3 A in the middle of the bilayer, with a profile closely resembling that of the disorder parameter. Our range of spherical radii is significantly narrower than the ranges reported by Ko¨nig et al. [63] for the bilayer at 30% hydra-
˚
tion, assuming a linear increase from the minimum to maximum values: 0.8–7.6 A from
˚
measurements with Q in the plane of the bilayer, and 1.1–5.8 A from measurements with Q along the bilayer normal. The origin of this discrepancy is difficult to ascertain without knowing the sensitivity of the fits to the data on the range and shape of the radius profile. To gain more insight it would be useful to see if the neutron data could be fit well using a variation of spherical radii resembling the profile in Figure 14b.
Although the diffusion-in-a-sphere model is illuminating in the sense that it conveys the notion of increasing dynamic disorder moving toward the bilayer interior, as a model for hydrocarbon chain dynamics it is not completely satisfying because it assumes independent motion for each proton and therefore lacks an explicit connection to conformational transitions. In this regard perhaps a more appealing model is the chain defect model introduced subsequently by Ko¨nig et al. [64] as an alternative to the diffusion-in-a-sphere model. In this model it is assumed that the primary mechanism for the proton jumps in the hydrocarbon chains detected by neutron time-of-flight measurements is the creation/ annihilation of a gtg′(g tg or g tg ) kink from/to an all-trans conformation. Two types of kink dynamics were considered. In the first, the kink is assumed to diffuse along the chains, whereas in the second a kink forms and disappears randomly at different points in the chains. Both models fit the data equally well, but the stochastic kink model is more consistent with the picture from MD simulations (for example, Fig. 8). The resulting model contains two parameters: the average number of gtg′ kinks per chain, 0.9, and the transition rate, r2 7.5 10 10 s 1, which corresponds to a kink lifetime of 1/r2 13 ps.
The chain defect model is useful because it incorporates a realistic mechanism for proton jumps into a model that leads to analytical expressions that can be used to fit neutron data. However, as pointed out by Ko¨nig et al., this model is an oversimplification, because clearly there are other types of chain defects (g, gg, g tg , and g tg ) that are forming and disappearing on the time scale of the experiment. Indeed, we find in our simulation that gtg′ kinks account for less than 20% of the gauche bonds in a fluid phase DPPC bilayer (Table 2). Moreover, we find that there are as many gtg (g tg or g tg ) kinks as gtg′ kinks. This is remarkable because a gtg kink produces a chain defect that significantly alters the path of the chain, whereas a gtg′ kink leaves the chain path largely unaltered. Consequently, a ttt to gtg transition is expected to lead to a larger displacement of more protons than a ttt to gtg′ transition. Thus, if our simulation results are trustworthy, we may conclude that the chain defect model including only gtg′ kinks significantly under-
Table 2 Conformational Defects in the Hydrocarbon
Chains in Fluid Phase Lipid Bilayers
Average number per chain
Type of defect |
MD |
Expt |
|
|
|
|
|
g |
3.6 |
3.7 [72], 3.6–4.2 [73] |
|
gtg |
0.35 |
1.2 |
[72] |
gtg gtg′ |
0.61 |
1.0 |
[74] |
|
|
|
|
Membrane Simulations |
491 |
estimates the proton motions arising from conformational transitions in the chains. The average total number of gauche bonds per chain in our simulation is in good agreement with estimates from FTIR spectra, but the numbers of kinks are significantly lower than the experimental estimates (Table 2). The seriousness of the discrepancy in the number of kinks is hard to assess because of uncertainties in assigning the spectra and the considerable disagreement between independent experimental measurements. We finish this section by noting that our simulation predicts a kink lifetime of 6 ps, which is about half that assumed in the chain defect model [64].
G.Water Dynamics
In contrast to the lipids, which can exhibit a wide variety of motions over a wide range of time scales, water dynamics in membranes on the time scale of tens of picoseconds are relatively uncomplicated, consisting of (ignoring biologically uninteresting high frequency vibrations) rigid-body translation and rotation. In this section we analyze the translational and rotational motion of water in multilamellar bilayers, highlighting the distinction between the bound and bulk waters identified earlier in Section III.C.
The center-of-mass mean-square displacements of the bulk and the three different classes of bound water molecules are plotted in Figure 15a. In all cases the MSDs display a linear time dependence beyond about 5 ps. Thus, the water translation at times longer than a few picoseconds can be described as Brownian motion, and the mobility can be quantified by a diffusion constant, D, proportional to the slope of the linear part of the MSD. The values obtained for motion in the plane and perpendicular to the plane of the bilayer, from the average of five 20 ps MSD increments, with the slopes calculated from 10 ps to 20 ps, are listed in Table 3. As expected, the bulk water molecules have the greatest translational mobility, followed by the choline-bound, phosphate-bound, and car- bonyl-bound water molecules. The tightly associated P-bound and CO-bound water molecules have roughly equal diffusion constants for in-plane and out-of-plane translational motion. The structural organization of the ‘‘bulk’’ waters that occupy a thin slab in the middle of the interlamellar space is quite similar to that of pure water at the same temperature, but the dynamics are different. As one might expect for water confined between two slabs (Fig. 2), we find that the diffusion constant in the plane of the bilayer is significantly larger ( 50%) than in the direction normal to the bilayer.
We discuss the rotational dynamics of water molecules in terms of the time correlation functions, Cl(t) Pl[cos θl(t)] (l 1, 2), where Pl is the lth Legendre polynomial, cos θl(t) ul(0) ul(t), u1, is a unit vector along the water dipole (HOH bisector), and u2 is a unit vector along an OH bond. Infrared spectroscopy probes C1(t), and deuterium NMR probes C2(t). According to the Debye model (Brownian rotational motion), both correlation functions are exponential, and C2(t) decays three times as fast as C1(t). The C1(t) for the different classes of water molecules, plotted in Figure 15b, display a rapid initial decay ( 10 ps) decay followed by a slower relaxation. The C2(t) (not shown) look similar but show a more rapid initial decay, as expected. Although the Cl(t) are better described by multiexponential or stretched exponential functions, we have estimated rotational correlation times by fitting the short-time (first 10 ps) decays to single exponentials, exp( t/τl) (Table 3). The trend in the rotational rates (1/τl) is the same as that in the translational diffusion constants, i.e., bulk bound, and the ratios τ1/τ2 are approximately 1.5 for all classes of water molecules, suggesting that the Debye model is not appropriate for describing the rotational motion of water molecules in the vicinity of membranes.
492 |
Tobias |
Figure 15 (a) Mean-square displacements of water molecules in three dimensions (see text for definitions of bound and bulk waters). (b) Time correlation functions for reorientation of the water OEH bonds.
We finish this section by comparing our results with NMR and incoherent neutron scattering experiments on water dynamics. Self-diffusion constants on the millisecond time scale have been measured by NMR with the pulsed field gradient spin echo (PFGSE) method. Applying this technique to oriented egg phosphatidylcholine bilayers, Wassall [68] demonstrated that the water motion was highly anisotropic, with diffusion in the plane of the bilayers hundreds of times greater than out of the plane. The anisotropy of
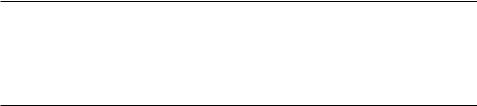
Membrane Simulations |
493 |
Table 3 Diffusion Constants and Rotational Correlation Times of Water Molecules from an MD Simulation of a Fully Hydrated Fluid Phase DPPC Bilayera
|
In-plane D |
Out-of-plane D |
Dipole τ1 |
OH bond τ2 |
Water class |
(10 5 cm2/s) |
(10 5 cm2/s) |
(ps) |
(ps) |
Bulk |
6.6 |
4.5 |
3.6 |
2.2 |
N-bound |
4.4 |
3.6 |
5.6 |
3.7 |
P-bound |
3.2 |
2.7 |
7.1 |
5.0 |
CO-bound |
2.3 |
2.0 |
9.1 |
5.9 |
a Note that in Ref. 55 the τ values for the P-bound and N-bound water molecules were erroneously interchanged. Source: Ref. 55.
the long-range diffusion observed on the microsecond time scale is consistent with our results but much more pronounced, as expected, given the disparity in time and length scales probed in the experiment and simulation. Wassall also observed that the diffusion constant increased with the number of water molecules per lipid molecule according to the Finer model of hydration shells [69] and consistent with the differences we found in the diffusion constants between the different classes of bound and bulk water [55].
An INS study of water dynamics in oriented DPPC bilayers was reported by Ko¨nig et al. [8]. Neutron time-of-flight measurements were performed on oriented samples at 44°C and two hydration levels, using a spectrometer with an energy resolution (0.015 meV) that corresponds to a maximum observable correlation time of a few hundred picoseconds. The spectral parameters are not available for comparison, so we compare parameters of the model used to fit the data, which includes bound water molecules that are assumed to undergo rotation diffusion only, and quasi-free water molecules that undergo both rotational and translational diffusion. At low hydration (three to four water molecules per lipid molecule) the data could be modeled as rotation only, with a rotational correlation time τR 60 ps. Apparently, this τR 1/DR, where DR is the rotational diffusion constant, should be divided by 6 for comparison with the simulation results in Table 2. The resulting 10 ps is close to our bound water values. To fit the data at higher hydration (11 waters per lipid), the parameters for the rotational motion were kept fixed and the translational motion was modeled as jump diffusion, giving a residence time of 2 ps and a diffusion constant D 1.6 10 5 cm2/s. Considering the 6°C difference in temperature, the diffusion constant extracted from the scattering data is close to the values we obtained for the most tightly bound waters (P- and CO-bound) in our simulation.
V.SUMMARY AND CONCLUSIONS
This chapter has given an overview of the structure and dynamics of lipid and water molecules in membrane systems, viewed with atomic resolution by molecular dynamics simulations of fully hydrated phospholipid bilayers. The calculations have permitted a detailed picture of the solvation of the lipid polar groups to be developed, and this picture has been used to elucidate the molecular origins of the dipole potential. The solvation structure has been discussed in terms of a somewhat arbitrary, but useful, definition of bound and bulk water molecules.
The majority of the chapter was focused on an analysis of dynamics, which to date