
J. Phys.: Condens. Matter 22 (2010) 346001 |
S Ghosh and B Sanyal |
were observed to be lower than the ferromagnetic one. Powder neutron diffraction experiments on Ni-excess and Mn-excess samples also observed similar behavior for the total magnetization. Furthermore, the neutron diffraction experiment also found that the magnetization is extremely sensitive to the chemical order in different sublattices of the disordered samples [9]. These two observations on total magnetization and chemical occupancy of sublattices demonstrate the need to investigate in detail the nature of magnetic interactions in the different sublattices and the role of chemical ordering to facilitate the microscopic understanding of the magnetic properties of off-stoichiometric NiMnGa alloys. There have been quite extensive theoretical studies on the nature of exchange interactions and hence the ordering temperatures in stoichiometric Heusler alloys [10]. Both the frozen magnon approach and real space based calculations of exchange parameters have been performed. Discussions have been made in terms of interand intra-sublattice exchange interactions between the magnetic atoms. However, to date, no investigation, theoretical or experimental, is available which shows the magnetic structures at different sublattices in a disordered sample, thereby interpreting the observed trends in magnetization in terms of direct evidence.
In this paper, we have studied the details of the magnetic interactions and the effect of chemical ordering on these interactions, and related them to the trends of total magnetization for three disordered NiMnGa alloys close to the stoichiometric composition. The neutron diffraction
results available |
for these |
three |
samples |
are interpreted |
by analyzing the |
results. |
We |
employ a |
first-principles |
electronic structure method in conjunction with a Heisenberg model to compute the sublattice specific pairwise exchange interactions. The exchange interactions can, both qualitatively and quantitatively, present the correct picture of the magnetic interactions. Using these results, we are able to explain the overall trends in the magnetization, thus providing a complete understanding of the magnetic properties in these systems as a function of composition and chemical ordering.
2. Computational details
The cubic phase of the alloys assumes an L21 (Heusler) structure [11]. In the stoichiometric composition of 50:25:25,
the sublattices (000) and ( 1 1 1 ) are occupied by Ni atoms
2 2 2
(sublattice I & IV), the sublattice ( 1 1 1 ) is occupied by Mn
4 4 4
atoms (sublattice II) and the sublattice ( 3 3 3 ) is occupied
4 4 4
by Ga atoms (sublattice III). For the off-stoichiometric alloys, if a sublattice is occupied by different atomic species, we assume that the atoms distribute randomly in the sublattice. To compute the exchange interactions in real space, we first performed electronic structure calculations using the Green’s function based Korringa–Kohn–Rostoker (KKR) method within the so called atomic sphere approximation (ASA) [12]. Chemical disorder has been treated by the coherent potential approximation (CPA). The Heisenberg pairexchange parameters were calculated using the theory of Lichtenstein et al [13], where the exchange interactions between a pair of spins is calculated using a classical
Heisenberg Hamiltonian. In this method, the exchange parameters, computed from the total energy variations due to the small rotations of a pair of spins causing a perturbation in spin density, are given by
|
|
|
|
1 |
|
EF |
|
|
||
|
|
Ji j |
= |
|
|
|
|
dE ImTrL ( i Tσi j j Tσj i ) |
(1) |
|
|
|
|
4π |
|
|
|||||
where |
i |
= |
t |
−1 |
− |
t |
−1 |
, σ the spin index, t the |
on-site |
|
|
|
i σ |
|
i σ |
|
|
scattering matrix and T is the scattering path operator related to the off-diagonal element of the Green function. TrL is the trace over the orbital indices of the scattering matrices. Positive (negative) values for Ji j indicate ferromagnetic (antiferromagnetic) coupling between atoms i and j .
Calculations have been performed for three off-stoichiom- etric alloys Ni1.968Mn1.22Ga0.812(Ni49), Ni2.008Mn1.16Ga0.832 (Ni50) and Ni2.08Mn1.04Ga0.88(Ni52) in their cubic austenite phases. These compositions were suggested by recent neutron diffraction experiments [9]. For the Ni49 and Ni50 samples, it was found in the modeling of data that by placing excess Mn in the vacant Ni and Ga sublattices for Ni49 and by placing excess Ni and excess Mn in the Ga sublattice for Ni50 produced a good fit to the observed diffraction pattern. For the Ni52 sample, which was excess in both Ni and Mn, placing the excess Mn and Ni on the Ga sublattice produced a poor fit to the diffraction pattern. An intuitive argument based upon elastic energies of the configurations was therefore used to model the occupancies by putting excess Ni on the Mn sublattice and excess Mn on the Ga sublattice. Such a model of chemical order improved the fit and model calculation of magnetization based upon this configuration (configuration 1) agreed with the measured value, while calculation using the previous configuration (configuration 2) predicted a large discrepancy between calculated and measured values.
3. Results and discussions
3.1. Magnetic moments
Our calculated magnetic moments for the above-mentioned compositions are shown in table 1. These moments have been calculated for the equilibrium volumes obtained from our calculations. For stoichiometric Ni2MnGa, Ni and Mn have local magnetic moments of 0.33 and 3.47 μB respectively. These numbers tally very well with the previously calculated ones. For all compositions in the off-stoichiometric alloys, Mn has a slightly reduced moment of around 3.3 μB in sublattice II. In sublattice III, even in the presence of Ga and some Ni, this local moment is sustained. However, for Ni49, Mn loses its moment significantly in the presence of a strong Ni environment in sublattice I. As usual, the Ni moment is observed to be very fragile in the presence of other specie. But it is slightly increased compared to stoichiometric Ni2MnGa. Ga has a small induced moment which is antiparallel to the Mn and Ni moments.
3.2. Interand intra-sublattice exchange interactions
In what follows, we compute the inter-sublattice and intrasublattice exchange parameters for various pairs of chemical
2
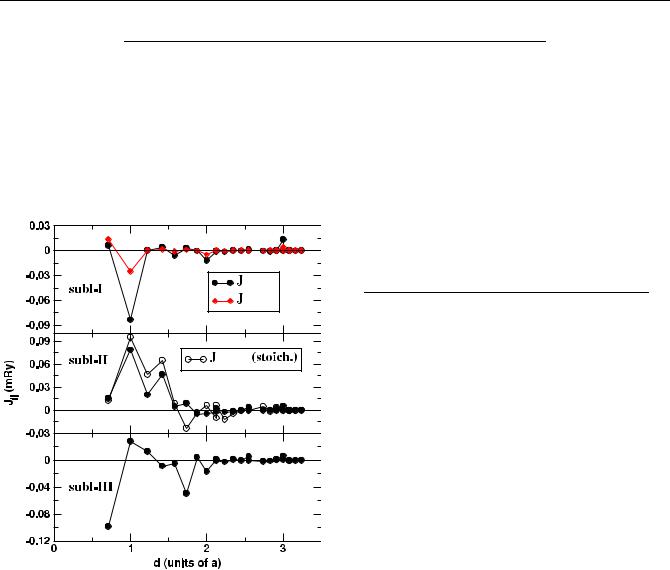
J. Phys.: Condens. Matter 22 (2010) 346001 S Ghosh and B Sanyal
Table 1. Configurations of various sublattices in Ni2MnGa, Ni49, Ni50 and Ni52 (both configurations Ni52I and Ni52II) along with the local magnetic moments (in Bohr-magnetons).
|
|
Sublattice I & IV |
Sublattice II |
Sublattice III |
|
|
|
|
|
Ni2MnGa |
Ni: 0.33 |
Mn: 3.47 |
Ga: −0.08 |
|
Ni49 |
|
Ni0.984Mn0.016 |
Mn |
Mn0.188Ga0.812 |
|
|
Ni: 0.43, Mn: −0.74 |
Mn: 3.31 |
Mn: 3.37, Ga: −0.07 |
Ni50 |
|
Ni |
Mn |
Ni0.008Mn0.16Ga0.832 |
Ni52 |
I |
Ni: 0.41 |
Mn: 3.32 |
Mn: 3.39, Ni: 0.09, Ga: −0.07 |
|
Ni |
Ni0.08Mn0.92 |
Mn0.12Ga0.88 |
|
Ni52 |
II |
Ni: 0.38 |
Ni: 0.16, Mn: 3.3 |
Mn: 3.36, Ga: −0.07 |
|
Ni |
Mn |
Ni0.08Mn0.04Ga0.88 |
|
|
|
Ni: 0.38 |
Mn: 3.29 |
Ni: 0.07, Mn: 3.36, Ga: −0.07 |
Mn-Mn |
Mn-Ni |
Mn-Mn |
Figure 1. Calculated Ji j s for Ni49 composition as a function of the separation between the pair of atoms: (top panel) JMn−Mn between Mn atoms, JMn−Ni between Ni atoms in sublattice I, (middle panel) JMn−Mn between Mn atoms in sublattice II in Ni49 as well as in stoichiometric Ni2MnGa, (bottom panel) JMn−Mn between Mn atoms in sublattice III. For explanation of nomenclature, readers are referred to table 1.
specie in these alloys. A complete understanding on the trends in magnetization can only be achieved when trends in both interand intra-sublattice interactions can be analyzed. It has been shown [14] that the magnetic properties in stoichiometric Ni2MnGa depends substantially on the inclusion of exchange interactions between all pairs of chemical components which are magnetic. Our analysis, thus focuses on the Ni–Ni, Ni–Mn and Mn–Mn interand intra-sublattice exchange parameters.
3.3. Ni49 and Ni50
In figure 1, we present the calculated intra-sublattice exchange interactions as a function of neighboring shells for Ni49. We focus on Mn–Mn and Ni–Mn interactions as these
Table 2. Inter-sublattice exchange interaction (in mRyd) between various chemical specie in different sublattices for the configurations described in table 1 and in the text. Ji indicate the exchange parameter for the i th neighbor.The blank spaces indicate that the relevant interactions are not possible.
Alloy |
Sublattice |
J1 |
J2 |
J3 |
J4 |
Ni49 |
Ni1–Mn2 |
0.50 |
0.005 |
0.00 |
0.00 |
|
Mn1–Mn2 |
0.95 |
−0.08 |
0.01 |
0.00 |
|
Ni1–Mn3 |
0.52 |
−0.01 |
0.00 |
0.00 |
|
Mn1–Mn3 |
0.92 |
0.02 |
−0.01 −0.02 |
|
|
Ni2–Mn3 |
— |
— |
— |
— |
|
Mn2–Mn3 |
−0.89 −0.09 |
−0.04 |
— |
|
Ni50 |
Ni1–Mn2 |
0.49 |
0.00 |
0.00 |
0.00 |
|
Mn1–Mn2 |
— |
— |
— |
— |
|
Ni1–Mn3 |
0.51 |
−0.01 |
0.00 |
0.00 |
|
Mn1–Mn3 |
— |
— |
— |
— |
|
Ni2–Mn3 |
— |
— |
— |
— |
|
Mn2–Mn3 |
−0.88 −0.09 |
−0.04 |
— |
|
Ni52I |
Mn2–Ni3 |
0.09 |
−0.04 |
0.00 |
— |
Ni1–Mn2 |
0.46 |
0.00 |
0.00 |
0.00 |
|
|
Mn1–Mn2 |
— |
— |
— |
— |
|
Ni1–Mn3 |
0.46 |
0.00 |
0.00 |
0.00 |
|
Mn1–Mn3 |
— |
— |
— |
— |
|
Ni2–Mn3 |
0.10 |
−0.05 |
0.00 |
— |
Ni52II |
Mn2–Mn3 |
−0.84 −0.1 |
−0.04 |
— |
|
Ni1–Mn2 |
0.44 |
0.00 |
0.00 |
0.00 |
|
|
Mn1–Mn2 |
— |
— |
— |
— |
|
Ni1–Mn3 |
0.47 |
−0.01 |
0.00 |
0.00 |
|
Mn1–Mn3 |
— |
— |
— |
— |
|
Ni2–Mn3 |
— |
— |
— |
— |
|
Mn2–Mn3 |
−0.84 −0.11 |
−0.04 |
— |
|
|
Mn2–Ni3 |
0.08 |
−0.04 |
0.00 |
— |
are supposed to be the catalysts for changes in overall magnetization. Mn atoms couple ferromagnetically in sublattice II where there is only Mn occupancy. Here we have also presented the calculated exchange interactions for the stoichiometric Ni2MnGa alloy. For the first few coordination shells, the strength of the ferromagnetic interaction between Mn atoms is larger in case of pure Ni2MnGa. It indicates that sublattice alloying decreases ferromagnetic order. The most interesting observation is that the quantitatively significant Mn–Mn interactions in sublattice I and III (up to sixth neighbor) are mostly antiferromagnetic with respect to the ones in sublattice II, the original Mn sublattice. The intersublattice exchange parameters are presented in table 2. The results suggest that the Mn atoms in sublattice II (the original
3
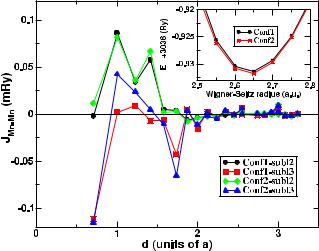
J. Phys.: Condens. Matter 22 (2010) 346001 |
S Ghosh and B Sanyal |
sublattice of Mn) couple antiferromagnetically with the Mn atoms in sublattice III while all other inter-sublattice Mn–Mn interactions are ferromagnetic. The interaction between Ni and Mn atoms in different sublattices are always ferromagnetic. The results for Ni50 follow the same trend. Quantitatively, the Ni–Mn interactions are much weaker than the Mn–Mn interactions. Thus, the overall trends in magnetization must be controlled by the Mn–Mn exchange interactions alone. The decrease in magnetization from Ni50 to Ni49, thus, can be attributed to the larger negative values in the firstneighbor Mn–Mn exchange parameter between sublattice II and sublattice III and in the larger negative values in the intrasublattice Mn–Mn exchange parameters in sublattice III in case of Ni49. This also confirms the proposition that the dominant Mn–Mn interactions between the Mn atoms in the original Mn sublattice and the Mn atoms in other sublattices in these alloys are antiferromagnetic and thereby, decrease the overall magnetization with respect to the stoichiometric composition. An interesting point to note is that in case of Ni49, the interactions between sublattice I and sublattice II as well as between sublattice I and sublattice III Mn atoms are ferromagnetic, with the nearest-neighbor exchange parameters being as large as those of the Mn–Mn sublattice II–sublattice III. However, the trends in magnetization are not affected by these interactions as sublattice I has an order of magnitude smaller amount of Mn in comparison to the other sublattices and thus the effective contribution from these interactions would be much smaller than the Mn2–Mn3 interactions. One may, thus, speculate about the presence of a competition between a Ruderman–Kittel–Kasuya–Yoshida- type ferromagnetic interaction and an antiferromagnetic superexchange interaction, as argued in the paper by Sasioglu et al [15]. For both alloys, there is no Ni–Ni intersublattice interactions, the intra-sublattice Ni–Ni interactions are much smaller in magnitude and therefore do not contribute substantially to the change in magnetization.
3.4. Ni52
To resolve the issue of ordering tendencies in the Ni52 sample and relate it to the magnetization, we first performed total energy calculations for configurations 1 and 2. The results are shown in the inset of figure 2. Although configuration 2 is energetically lower than configuration 1, the energy difference is less than 1 meV/f.u., signifying that both configurations are equally probable. The results in table 2 suggest that the inter-sublattice Mn–Mn interactions (between Mn atoms in sublattice II and in sublattice III) are qualitatively as well as quantitatively the same for both configurations. The same goes for the Ni–Mn exchange parameters. However, the intra-sublattice exchange interaction between Mn atoms are substantially different for the two configurations, as shown in figure 2. The Mn atoms in the original Mn sublattice couple ferromagnetically for both configurations. The quantitative and qualitative variation of the exchange parameters with neighboring shells are almost the same for both configurations. The only significant differences are in the first and fourth shell parameters. For configuration 1, the nearest-neighbor
tot |
Figure 2. Calculated Ji j s for Ni52 composition in two different configurations as a function of the separation between the atoms i and j . Parameters for sublattice 2 and 3 of configuration 1 are denoted as circles and squares respectively whereas the same for configuration 2 are indicated as diamonds and triangles respectively. (Inset) Total energies as a function of Wigner–Seitz radii for the two configurations.
exchange parameter is negative, while for configuration 2, it is positive. However, the values are close to 0. The fourthneighbor parameters for configuration 1 are slightly smaller than the ones corresponding to configuration 2, thus lowering the Mn moment in the original sublattice in configuration 1 with respect to that of configuration 2. The variations of intra-sublattice exchange parameters for both configurations are almost identical to that of Ni49, indicating that the onsite Mn interactions do not change significantly upon changing
composition and |
sublattice |
occupancies. |
The situation |
is quite different |
with the |
off-site Mn–Mn interactions. |
For configuration 1, where the sublattice III is occupied with Mn and Ga atoms, the Mn–Mn coupling is mostly antiferromagnetic. For the second and third neighboring shells, the coupling is ferromagnetic but the quantitative values are much smaller than the antiferromagnetic ones. In case of configuration 2, on the other hand, where the sublattice III is occupied by Ni, Mn and Ga atoms, there are significantly large positive values of Mn–Mn exchange parameters for the second and third neighbors. Thus, one can conclude that the net magnetic moment for configuration 1 is smaller than that of the configuration 2 due to the larger negative values of the sublattice III Mn–Mn interactions. This is in accordance with the experimental observation that the magnetic moment calculated with configuration 1 is much smaller than that obtained with configuration 2 and is closer to the measured value. The results on exchange interactions, therefore, validate the proposition that the correct ordering in Ni52 is given by configuration 1.
The decrease in magnetic moment as one goes from Ni52 to Ni49, in spite of the fact that the Mn concentration increases from 1.04 to 1.22, as is observed experimentally (the moment per formula unit is 3.64 μB in Ni52 and 3.44 μB in Ni49), can thus be explained from the Mn–Mn interactions (both
4