
- •The Acoustical Society of America
- •Preface
- •Contents
- •About the Authors
- •1 Introduction
- •1.2 Specialized Areas within Acoustics
- •1.3 About the History of Acoustics
- •1.4 Relevant Quantities in Acoustics
- •1.5 Some Numerical Examples
- •1.6 Logarithmic Level Ratios and Logarithmic Frequency Ratios
- •1.7 Double-Logarithmic Plots
- •1.8 Exercises
- •2 Mechanic and Acoustic Oscillations
- •2.1 Basic Elements of Linear, Oscillating, Mechanic Systems
- •2.2 Parallel Mechanic Oscillators
- •2.3 Free Oscillations of Parallel Mechanic Oscillators
- •2.4 Forced Oscillation of Parallel Mechanic Oscillators
- •2.5 Energies and Dissipation Losses
- •2.6 Basic Elements of Linear, Oscillating, Acoustic Systems
- •2.7 The Helmholtz Resonator
- •2.8 Exercises
- •3.1 The Electromechanic Analogies
- •3.2 The Electroacoustic Analogy
- •3.3 Levers and Transformers
- •3.4 Rules for Deriving Analog Electric Circuits
- •3.5 Synopsis of Electric Analogies of Simple Oscillators
- •3.6 Circuit Fidelity, Impedance Fidelity and Duality
- •3.7 Examples of Mechanic and Acoustic Oscillators
- •3.8 Exercises
- •4.2 The Carbon Microphone—A Controlled Coupler
- •4.3 Fundamental Equations of Electroacoustic Transducers
- •4.4 Reversibility
- •4.5 Coupling of Electroacoustic Transducers to the Sound Field
- •4.6 Pressure and Pressure-Gradient Receivers
- •4.7 Further Directional Characteristics
- •4.8 Absolute Calibration of Transducers
- •4.9 Exercises
- •5 Magnetic-Field Transducers
- •5.1 The Magnetodynamic Transduction Principle
- •5.2 Magnetodynamic Sound Emitters and Receivers
- •5.3 The Electromagnetic Transduction Principle
- •5.4 Electromagnetic Sound Emitters and Receivers
- •5.5 The Magnetostrictive Transduction Principle
- •5.6 Magnetostrictive Sound Transmitters and Receivers
- •5.7 Exercises
- •6 Electric-Field Transducers
- •6.1 The Piezoelectric Transduction Principle
- •6.2 Piezoelectric Sound Emitters and Receivers
- •6.3 The Electrostrictive Transduction Principle
- •6.4 Electrostrictive Sound Emitters and Receivers
- •6.5 The Dielectric Transduction Principle
- •6.6 Dielectric Sound Emitters and Receivers
- •6.7 Further Transducer and Coupler Principles
- •6.8 Exercises
- •7 The Wave Equation in Fluids
- •7.1 Derivation of the One-Dimensional Wave Equation
- •7.3 Solutions of the Wave Equation
- •7.4 Field Impedance and Power Transport in Plane Waves
- •7.6 The Acoustic Measuring Tube
- •7.7 Exercises
- •8 Horns and Stepped Ducts
- •8.2 Conical Horns
- •8.3 Exponential Horns
- •8.4 Radiation Impedances and Sound Radiation
- •8.5 Steps in the Area Function
- •8.6 Stepped Ducts
- •8.7 Exercises
- •9.1 The Spherical Wave Equation
- •9.2 Spherical Sound Sources of the First Order
- •9.3 Spherical Harmonics
- •9.4 Higher-Order Spherical Sound Sources
- •9.5 Line Arrays of Monopoles
- •9.6 Analogies to Fourier Transform in Signal Theory
- •9.7 Directional Equivalence of Sources and Receivers
- •9.8 Exercises
- •10.1 The Rayleigh Integral
- •10.3 The Far-Field of Piston Membranes
- •10.4 The Near-Field of Piston Membranes
- •10.5 General Remarks on Diffraction and Scattering
- •10.6 Exercises
- •11.1 Dissipation During Sound Propagation in Air
- •11.2 Sound Propagation in Porous Media
- •11.4 Wall (Surface) Impedance and Degree of Absorption
- •11.5 Porous Absorbers
- •11.6 Resonance Absorbers
- •11.7 Exercises
- •12.1 Mirror Sound Sources and Ray Tracing
- •12.2 Flutter Echoes
- •12.3 Impulse Responses of Rectangular Rooms
- •12.4 Diffuse Sound Fields
- •12.5 Reverberation-Time Formulas
- •12.6 Application of Diffuse Sound Fields
- •12.7 Exercises
- •13.1 Sound in Solids—Structure-Borne Sound
- •13.2 Radiation of Airborne Sound by Bending Waves
- •13.3 Sound-Transmission Loss of Single-Leaf Walls
- •13.4 Sound-Transmission Loss of Double-Leaf Walls
- •13.5 The Weighted Sound-Reduction Index
- •13.6 Insulation of Vibrations
- •13.7 Insulation of Floors with Regard to Impact Sounds
- •13.8 Exercises
- •14 Noise Control—A Survey
- •14.1 Origins of Noise
- •14.2 Radiation of Noise
- •14.3 Noise Reduction as a System Problem
- •14.4 Noise Reduction at the Source
- •14.5 Noise Reduction Along the Propagation Paths
- •14.6 Exercises
- •15 Solutions to the Exercise Problems
- •16 Appendices
- •16.1 Complex Notation of Sinusoidal Signals
- •16.2 Complex Notation of Power and Intensity
- •16.3 Supplementary Textbooks for Self Study
- •16.4 Letter Symbols, Notations, and Units
- •Index

10 |
|
|
|
|
|
|
|
|
1 |
Introduction |
|
Table 1.3 Frequencies versus corresponding wavelengths in air |
|
|
|
|
|||||||
|
|
|
|
|
|
|
|
|
|
|
|
Octave-center |
16 |
32 |
63 |
125 |
250 |
500 |
1k |
2k |
4k |
8k |
16k |
frequency [Hz] |
|
|
|
|
|
|
|
|
|
|
|
|
|
|
|
|
|
|
|
|
|
|
|
Wave length |
20 |
10 |
5 |
2.5 |
1.25 |
0.63 |
0.32 |
0.16 |
0.08 |
0.04 |
0.02 |
in air [m] |
|
|
|
|
|
|
|
|
|
|
|
|
|
|
|
|
|
|
|
|
|
|
|
Ψsemitone = 12 ld ( f2/ f1), |
in [semitone] |
Ψcent = 1200 ld ( f2/ f1), |
in [cent] |
The first two logarithmic frequency intervals are often called octave and thirdoctave. The equations, Ψoct = 1, indicates one octave. Ψ3rd oct = 1 indicates one third of an octave. The four logarithmic frequency intervals have the following relationship to each other, 1 oct = 3·3rd oct = 12 semitone = 1200 cent.
A bandpass filter, which is a filter that filters out certain regions from a frequency spectrum and blocks the rest, is called octave filter when the difference between the upper and lower limiting frequencies of the pass-band amounts to one octave. Accordingly, there are, for example, third-octave filters. Note that the specification of limiting frequencies is task-specific.
In communication engineering, decades (10 : 1) are sometimes preferred to octaves (2 : 1). Conversion is as follows: 1 oct ≈ 0.3 dec or 1 dec ≈ 3.3 oct.
Wavelength, λ, and frequency, f , of an acoustic wave are linked by the relationship c = λ · f . In air we have c ≈ 340 m/s. In Table 1.3, a series of frequencies is presented with their corresponding wavelengths in air. The series is taken from a standardized octave series that is recommended for use in engineering acoustics.
In the audible frequency range, the wavelengths extend from a few centimeters to many meters. Because radiation, propagation, and reception of waves are characterized by the linear dimension of reflecting surfaces relative to the wavelength, a wide variety of different effects, including reflection, scattering, and diffraction, are experienced in acoustics.
1.7 Double-Logarithmic Plots
By plotting levels over logarithmic frequency intervals, one obtains a doublelogarithmic graphic representation of the original quantities. This way of plotting has some advantages over linear representations and is quite popular in acoustics.7 Figure 1.2a presents an example of a linear representation, and Fig. 1.2b shows its corresponding double-logarithmic plot.
In double-logarithmic plots, all functions that are proportional to ω y appear as straight lines since
7 In network theory, double-logarithmic graphic representations are known as Bode diagrams.
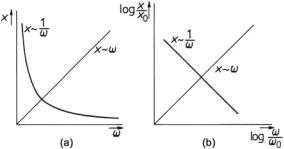
1.7 Double-Logarithmic Plots |
11 |
Fig. 1.2 Different representations of frequency functions. (a) Linear. (b) Double-logarithmic
x = a ω y → log x = log a + y log |
ω . |
(1.13) |
For integer potencies, y = ± n with n = 1, 2, 3, . . ., |
we arrive |
at slopes of |
± n · 6 dB/oct for sound pressure, displacement, and particle velocity, and of ± n · 3 dB/oct for power and intensity. For decades the respective values are ≈20 dB/dec and ≈10 dB/dec.
Functions with different potency of ω are quite frequent in acoustics. This results from the fact that differential equations of different degrees are used to describe vibrations and waves. The slope of the lines in the plot helps to estimate the order of the underlying oscillation processes.
1.8 Exercises
Recapitulation of Complex Notation
Problem 1.1 Use Euler’s formula to derive a real-valued sinusoidal expression for z(t) from the complex-valued exponential time function. Euler’s formula reads as follows,
|
= ˆ |
|
z |
z e j (ω t+φ). |
(1.14) |
Problem 1.2 Given a sinusoidal electric voltage signal
u(t) = uˆ cos(ω t + φ) , |
(1.15) |
find the electric current, i(t), in Fig. 1.3 by applying complex notation.
Problem 1.3 For experimental determination of the mechanic impedance, Z mech, of a solid material (object) in practice, one may excite the object under test by
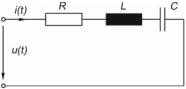
12 |
1 Introduction |
Fig. 1.3 Serial electric circuit consisting of resistance, R, inductance, L, and capacitance, C
applying a sinusoidal |
force, F(t) = |
F cos( |
ω |
t |
+ φF |
), and measuring the accelera- |
|
ˆ |
|
|
tion, a(t) = aˆ cos(ω t + φa), at the point of interest.
Determine the mechanic impedance, Z mech, at this point via the given excitation and the resulting acceleration responses.
Problem 1.4 Given a reference pressure of p0, rms = 20 µN/m2,
(a)Find the sound-pressure level of prms = 0.1 N/m2 and prms = 102 N/m2, respectively.
(b)Evaluate the sound-pressure levels for the case that the sound pressures under (a) are three-times larger.
Problem 1.5 Which frequency ratios and which logarithmic frequency intervals are represented by three semitones and by twelve semitones?
Problem 1.6Ψ |
oct = ld ( f2/ f1), |
oct |
] |
|
|
in [1 |
|
||
Ψ1/3rd oct = 3 ld ( f2/ f1), |
in [ 3 |
oct] |
||
Ψsemitone = 12 ld ( f2/ f1), |
in [semitone] |
|||
Ψcent = 1200 ld ( f2/ f1), |
in [cent] |
Given the third-octave-band interval, Ψ3rd oct = 1, and the octave-band interval,
Ψoct = 1.
–Determine the corresponding frequency ratios, ( f2/ f1)3rd oct and ( f2/ f1)oct.
–Use these frequency ratios to find the two limiting frequencies, that is, the lower limiting and the upper limiting frequency, fl, and fu, of a 3rd-octave band and of an octave band with a given center frequency of fc.
Problem 1.7 Establish a table for the addition of sound-pressure levels of multiple incoherent sound sources of equal level and equal distance to the receiving point. The number of sound sources is 1, 2, 3, 4, 5, 6, 8, or 10, repectively.
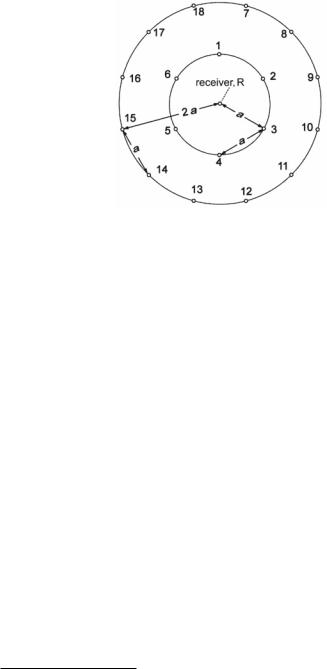
1.8 Exercises |
13 |
Fig. 1.4 Multiple sound sources of equal intensity in an open-space office
Problem 1.8 In an open-space office there are multiple noise sources (talking persons), spatially distributed as shown in Fig. 1.4. Sources 1–6 are located along a circle of radius a around the receiver, R, with a mutual distance of a between them. Sources 7–18 are located apart from each other by, again, a distance of a and along a circle of radius 2 a around the receiver. The sound-pressure level is assumed to decrease by 5 dB when doubling the distance in this particular space.8
With the sound-pressure level being 65 dB(A) at a 1-m distance from any single source (talker) for all directions, find the sound-pressure level at the receiver as a function of the number of sound sources (a = 4 m).
8 This, by the way, indicates that the sound-field in the specific space given here is not an entirely free field. For a perfectly free-field, we would expect a decrease of 6 dB/distance-doubling for spherical sound sources (4.25).