
- •The Acoustical Society of America
- •Preface
- •Contents
- •About the Authors
- •1 Introduction
- •1.2 Specialized Areas within Acoustics
- •1.3 About the History of Acoustics
- •1.4 Relevant Quantities in Acoustics
- •1.5 Some Numerical Examples
- •1.6 Logarithmic Level Ratios and Logarithmic Frequency Ratios
- •1.7 Double-Logarithmic Plots
- •1.8 Exercises
- •2 Mechanic and Acoustic Oscillations
- •2.1 Basic Elements of Linear, Oscillating, Mechanic Systems
- •2.2 Parallel Mechanic Oscillators
- •2.3 Free Oscillations of Parallel Mechanic Oscillators
- •2.4 Forced Oscillation of Parallel Mechanic Oscillators
- •2.5 Energies and Dissipation Losses
- •2.6 Basic Elements of Linear, Oscillating, Acoustic Systems
- •2.7 The Helmholtz Resonator
- •2.8 Exercises
- •3.1 The Electromechanic Analogies
- •3.2 The Electroacoustic Analogy
- •3.3 Levers and Transformers
- •3.4 Rules for Deriving Analog Electric Circuits
- •3.5 Synopsis of Electric Analogies of Simple Oscillators
- •3.6 Circuit Fidelity, Impedance Fidelity and Duality
- •3.7 Examples of Mechanic and Acoustic Oscillators
- •3.8 Exercises
- •4.2 The Carbon Microphone—A Controlled Coupler
- •4.3 Fundamental Equations of Electroacoustic Transducers
- •4.4 Reversibility
- •4.5 Coupling of Electroacoustic Transducers to the Sound Field
- •4.6 Pressure and Pressure-Gradient Receivers
- •4.7 Further Directional Characteristics
- •4.8 Absolute Calibration of Transducers
- •4.9 Exercises
- •5 Magnetic-Field Transducers
- •5.1 The Magnetodynamic Transduction Principle
- •5.2 Magnetodynamic Sound Emitters and Receivers
- •5.3 The Electromagnetic Transduction Principle
- •5.4 Electromagnetic Sound Emitters and Receivers
- •5.5 The Magnetostrictive Transduction Principle
- •5.6 Magnetostrictive Sound Transmitters and Receivers
- •5.7 Exercises
- •6 Electric-Field Transducers
- •6.1 The Piezoelectric Transduction Principle
- •6.2 Piezoelectric Sound Emitters and Receivers
- •6.3 The Electrostrictive Transduction Principle
- •6.4 Electrostrictive Sound Emitters and Receivers
- •6.5 The Dielectric Transduction Principle
- •6.6 Dielectric Sound Emitters and Receivers
- •6.7 Further Transducer and Coupler Principles
- •6.8 Exercises
- •7 The Wave Equation in Fluids
- •7.1 Derivation of the One-Dimensional Wave Equation
- •7.3 Solutions of the Wave Equation
- •7.4 Field Impedance and Power Transport in Plane Waves
- •7.6 The Acoustic Measuring Tube
- •7.7 Exercises
- •8 Horns and Stepped Ducts
- •8.2 Conical Horns
- •8.3 Exponential Horns
- •8.4 Radiation Impedances and Sound Radiation
- •8.5 Steps in the Area Function
- •8.6 Stepped Ducts
- •8.7 Exercises
- •9.1 The Spherical Wave Equation
- •9.2 Spherical Sound Sources of the First Order
- •9.3 Spherical Harmonics
- •9.4 Higher-Order Spherical Sound Sources
- •9.5 Line Arrays of Monopoles
- •9.6 Analogies to Fourier Transform in Signal Theory
- •9.7 Directional Equivalence of Sources and Receivers
- •9.8 Exercises
- •10.1 The Rayleigh Integral
- •10.3 The Far-Field of Piston Membranes
- •10.4 The Near-Field of Piston Membranes
- •10.5 General Remarks on Diffraction and Scattering
- •10.6 Exercises
- •11.1 Dissipation During Sound Propagation in Air
- •11.2 Sound Propagation in Porous Media
- •11.4 Wall (Surface) Impedance and Degree of Absorption
- •11.5 Porous Absorbers
- •11.6 Resonance Absorbers
- •11.7 Exercises
- •12.1 Mirror Sound Sources and Ray Tracing
- •12.2 Flutter Echoes
- •12.3 Impulse Responses of Rectangular Rooms
- •12.4 Diffuse Sound Fields
- •12.5 Reverberation-Time Formulas
- •12.6 Application of Diffuse Sound Fields
- •12.7 Exercises
- •13.1 Sound in Solids—Structure-Borne Sound
- •13.2 Radiation of Airborne Sound by Bending Waves
- •13.3 Sound-Transmission Loss of Single-Leaf Walls
- •13.4 Sound-Transmission Loss of Double-Leaf Walls
- •13.5 The Weighted Sound-Reduction Index
- •13.6 Insulation of Vibrations
- •13.7 Insulation of Floors with Regard to Impact Sounds
- •13.8 Exercises
- •14 Noise Control—A Survey
- •14.1 Origins of Noise
- •14.2 Radiation of Noise
- •14.3 Noise Reduction as a System Problem
- •14.4 Noise Reduction at the Source
- •14.5 Noise Reduction Along the Propagation Paths
- •14.6 Exercises
- •15 Solutions to the Exercise Problems
- •16 Appendices
- •16.1 Complex Notation of Sinusoidal Signals
- •16.2 Complex Notation of Power and Intensity
- •16.3 Supplementary Textbooks for Self Study
- •16.4 Letter Symbols, Notations, and Units
- •Index
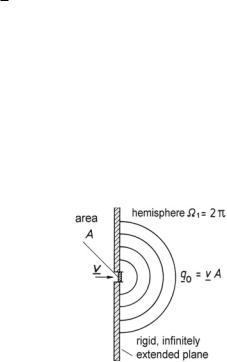
154 |
10 Piston Membranes, Diffraction and Scattering |
10.1 The Rayleigh Integral
A small vibrating piston results in a 1st-order spherical sound field—see Fig. 9.6b. However, when hydrodynamic shorting between the front and back of the baffle is prohibited by placing this piston into an infinitely extended, plane and rigid baffle, we get a hemispherical sound field of 0th order radiating into half of the space— illustrated in Fig. 10.1. The hemispherical sound field is
|
→(r) = |
j ω |
e−jβr |
|
(10.1) |
||||
p |
|
|
q |
0 |
|
, |
|||
2 π |
r |
||||||||
|
|
|
|
where 2π is the spatial angle of a hemisphere. Assuming that the baffle is flat, this sound field has no normal components in the baffle’s plane, and, therefore, the baffle cannot cause any reflection or diffraction.
Now consider an oscillating membrane with an area A0 in this infinitely extended, flat and plane baffle where all area elements oscillate perpendicularly to the area. We consider this arrangement the superposition of an infinite number of adjacent monopoles, dq 0 = v d A,—depicted in Fig. 10.2. The total sound pressure at an observation point is found by superposition of these monopoles, vis,
p |
→(r) = |
j ω |
|
v(x, y) |
e−jβr |
d A . |
(10.2) |
|
2 π |
A0 |
r |
||||||
|
|
|
|
In acoustics, this integral is usually called Rayleigh integral. It is valid at all distances from the membrane.
It is worth noting that v (x, y) needs not be the same everywhere on the membrane. Its value may depend on the position of the membrane. A finite area A0 is required for the Rayleigh integral to converge without additional assumptions, such as propagation losses in the medium. Further, no obstacles are admitted in the hemisphere concerned.
Fig. 10.1 Hemispherical sound field, originating from a point source in a flat and rigid baffle
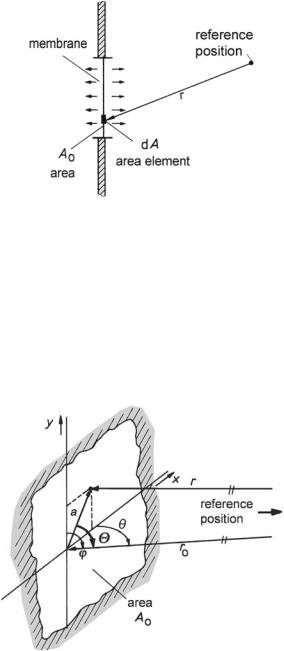
10.2 Fraunhofer’s Approximation |
155 |
Fig. 10.2 Sound field in front of a membrane in a flat and rigid baffle
10.2 Fraunhofer’s Approximation
Fraunhofer’s approximation applies when the distance from the reference point to the membrane is very large compared to the linear dimensions of the radiating membrane. Figure 10.3 depicts the situation to be discussed.
The quantity, r0, is the distance from the reference point to any position on the membrane within the area A0. That position is preferably the membrane’s center of
Fig. 10.3 Fraunhofer’s approximation
156 |
10 Piston Membranes, Diffraction and Scattering |
gravity. The angle between r and r0 is very small, and the two lines are practically parallel. The following linear approximation is thus applicable,
r ≈ r0 − a cos Θ , |
(10.3) |
where Θ is the angle between r0 and a. |
|
We express this setting in Cartesian coordinates as |
|
r ≈ r0 − x cos θ − y cos ϕ , |
(10.4) |
where θ is the angle between r0 and the x-axis, and ϕ is the angle between r0 and the y-axis. The factor 1/r is brought out in front of the integral because 1/r ≈ 1/r0—as we saw in Sect. 9.5. Thus, we get
p |
→(r, θ, ϕ) ≈ |
j ω e−jβr0 |
× |
|
2 π r0 |
||||
|
+∞ +∞
v(x, y) e j(β cos θ)x e j(β cos ϕ)y dx dy . (10.5)
−∞ −∞
This expression is again isomorphic to a Fourier transform. It is actually a twodimensional, spatial Fourier transform, where β cos θ is the phase coefficient in x- direction, and β cos ϕ is the phase coefficient in y-direction. Recall that β = 2π/λ.
10.3 The Far-Field of Piston Membranes
This section deals with the sound field produced by rigid membranes, so-called piston membranes, in infinitely extended, rigid, flat baffles. In other words, we speak of baffled pistons with identical v (x, y) everywhere on the piston. Such piston membranes qualify as appropriate models for loudspeakers in large baffles as long as the membranes of the loudspeakers vibrate in phase.
Rectangular Piston Membranes
The computation of the sound field of a rectangular piston membrane in an infinitely extended, rigid, flat baffle is feasible with Fraunhofer’s approximation according to Fig. 10.4. By symbolizing the constant factors left of the integral as C 1 or C 2, respectively, we write Fraunhofer’s approximation and its Fourier transform as follows,
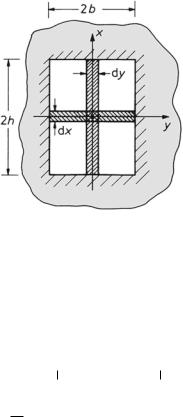
10.3 The Far-Field of Piston Membranes |
157 |
Fig. 10.4 Rectangular membrane
p →(r, θ, ϕ) = C 1 |
+b |
|
+h |
v e j(β cos θ)x e j(β cos ϕ)y dx dy |
(10.6) |
|||||||||||||||||
b |
|
h |
||||||||||||||||||||
|
|
|
|
− |
|
|
− |
|
|
|
|
|
|
|
|
|
|
|
|
|
|
|
|
|
|
= C 2 |
+h |
|
|
|
|
|
|
|
|
+b |
|
|
|
|
|
|
|
|
|
|
|
|
e j(β cos θ)x dx |
e j(β cos ϕ)y dy , |
(10.7) |
|||||||||||||||||
|
|
|
|
−h |
|
|
|
|
|
|
|
|
−b |
|
|
|
|
|
|
|
|
|
|
|
|
|
|
|
◦ |
|
|
|
|
|
|
|
|
◦ |
|
||||||
|
|
|
|
|
|
• |
|
|
|
|
|
|
|
|
• |
|
||||||
|
p |
→(r, θ, ϕ) ◦ |
• C 2 2h si (h β cos θ) 2b si (b β cos ϕ) . |
(10.8) |
||||||||||||||||||
|
|
|
||||||||||||||||||||
|
|
|
|
|
|
|
|
|
|
|
|
|
|
|
|
|
|
|
|
|||
|
|
|
|
|
|
|
|
|
Γ (θ) |
|
|
|
Γ (ϕ) |
|
Because each of the two integrals is a one-dimensional Fourier integral, the integration is subject to well-known rules. The result of the integration is (10.8), where the first term on the right-hand side includes the directional characteristic concerning θ, and the second one for ϕ. The total two-dimensional directional characteristic is formed by multiplying the two one-dimensional characteristics. Each of them represents a continuously loaded line array, one on the x-axis, and another on the y-axis. Thus, we get
Γ (θ, ϕ) = Γ (θ) Γ (ϕ) . |
(10.9) |
The third dimension, r, is not considered because the derivation above only deals with the sound field far away from the membrane.
Circular Piston Membranes
The calculation of circular pistons is slightly more complicated and requires a transformation into polar coordinates—illustrated in Fig. 10.5. The result of the calculation is given below,