
Molecular Sieves - Science and Technology - Vol. 6 - Characterization II / 04-NMR of Physisorbed 129Xe Used as a Probe to Investigate Molecular Sieves
.pdf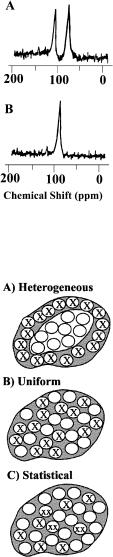
NMR of Physisorbed 120XE Used as a Probe to Investigate Molecular Sieves |
195 |
Fig. 19 129Xe NMR spectra of Xe (PXe = 40 kPa) adsorbed in NaY zeolite with an average loading corresponding to 0.5 HMB molecule/supercage. A: before heating; B: after heating at 573 K for 2 h. (Reprinted with permission from [161]. Copyright (1987) American Chemical Society)
Fig. 20 Possible dispersion of guest molecules among cavities of a zeolite particle when the average coverage is less than one molecule per cavity. (Reprinted with permission from [161]. Copyright (1987) American Chemical Society)
that the guest molecules were then homogeneously distributed among the zeolite cavities.
This is consistent with the distributions shown in Fig. 20: 50% of supercages are occupied by one HMB molecule and 50% are free of HMB
(B) or there is a statistical distribution (C). Both are macroscopically uni-

196 |
J.-L. Bonardet et al. |
form in the zeolite, and both would yield only one average xenon NMR line. Unfortunately, because of the high xenon mobility the resonance line is averaged and the two distributions cannot be distinguished by the 129Xe NMR technique alone. Nevertheless, with additional information from multiplequantum NMR spectroscopy, it is possible to conclude unambiguously that the distribution inside the supercages is homogeneous.
The same authors [71, 163] extended their study to other molecules: n- hexane, benzene and 1,3,5-trimethylbenzene (TMB). Table 5 presents values of δ for PXe = 0 and the slope of the δ = f (N) curve when the xenon concentration is 0, 1 or 2 molecules per supercage. The samples with adsorbed benzene and TMB show similar values of δ at all concentrations of guest species and with n-hexane the largest values of the chemical shift. Certain conclusions can be drawn regarding the location of the guest molecules inside the supercages. As suggested by Lechert et al. [164] and by de Mallmann and Barthomeuf [165], benzene is probably “attached” to the walls of the supercages near cation sites. Since the geometry of TMB is similar to that of benzene and has the same chemical shift at zero xenon pressure, it may be attached in a similar manner. The much larger interaction between xenon and n-hexane is attributed to the fact that the molecules have no preference for being attached parallel or perpendicular to the supercage surface and, therefore, protrude into the center of the cage allowing a greater interaction. Figure 21 resumes the different situations allowed for adsorbed molecules.
The variation of the slope of the δ = f (N) variation is related either to the void volume or to the channel blockage. For the three benzene samples, the similarity of the slopes indicates that benzene does not interfere with xenon
Table 5 δN →0 and slope of the δ = f (N) variations for different guest molecules at various loadings
Guest molecules |
Molecules/cage |
δ at N = 0 |
Slope δ = f (N) |
|
|
(ppm) |
(ppm/1020 Xe g–1 of NaY) |
|
|
|
|
|
2 |
89.7 ± 0.1 |
3.8 ± 0.1 |
benzene |
1 |
75.1± 1.1 |
3.9 ± 0.2 |
|
0.5 |
68.6 ± 0.1 |
4.0 ± 0.1 |
|
2 |
89.0 ± 1.3 |
5.4 ± 0.3 |
trimethylbenzene |
1 |
75.9 ± 0.4 |
4.5 ± 0.1 |
|
0.5 |
71.2 ± 0.7 |
3.6 ± 0.1 |
|
2 |
1 07.7 ± 1.2 |
4.4 ± 0.3 |
n-hexane |
1 |
86.7 ± 0.8 |
3.4 ± 0.2 |
|
0.5 |
74.7 ± 0.5 |
3.5 ± 0.1 |
none (this work) |
|
58.9 ± 0.5 |
4.4 ± 0.1 |
none (ref. 1) |
|
58 ± 4 |
4.6 ± 0.2 |

NMR of Physisorbed 120XE Used as a Probe to Investigate Molecular Sieves |
197 |
Fig. 21 Schematic illustration of the various guest species adsorbed inside the NaY zeolite supercages. Two n-hexane molecules adsorbed: normal to the supercage (A); parallel to the supercage (B); two benzene molecules (C); two TMB molecules (D). (Reprinted with permission from [163]. Copyright (1990) American Chemical Society)
by taking up volume in the middle of the supercages or by blocking the channels. For TMB, the situation is not so clear. If TMB adsorbs like benzene, it would block the channels of the zeolite because of its larger kinetic diameter (0.75 nm) and then interfere with xenon atoms. On the other hand, if it did not attach to the supercage walls, it could occupy a large fraction (up to 40% for 2 molecules per supercage) of the void volume, but in both cases the slope would increase with the xenon concentration. It is difficult to explain the n-hexane data: one reason for the larger slope, observed only at a concentration of two n-hexane molecules, may be that the probability of having the 2 molecules normal to the surface is greater than when the molecules are attached like benzene and TMB, near the SII sites (Fig. 21A). In this case, interaction with xenon is enhanced.
Liu and coworkers [166, 167] investigated in more detail the distribution of benzene in Na – X and Na – Y zeolites. First of all, they showed that benzene diffuses very slowly at room temperature in the zeolite crystallites. Even after 120 days, adsorption equilibrium is not reached and it is necessary to heat the sample to 523 K for 10 h to obtain a homogeneous distribution.
Moreover, the variation of the 129 Xe NMR linewidth, ∆H, with the average number of benzene molecules per cage, θ, shows an unexpected marked increase for 2.5 < θ < 3.5 (Fig. 22). The small linear increase in ∆H with θ (for θ < 2.5) is in agreement with the decrease in the mean free path of xenon proposed by Demarquay and Fraissard [56], and is due either to a decrease in the void space available to the xenon atoms or to an increase in site-hopping motion of the adsorbed benzene molecules. The fact that the linewidths are nearly independent of the xenon concentration proves that xenon atoms can move rapidly between supercages and that the effects of Xe – Xe interactions are reduced. For 2.5 < θ < 3.5, the ∆H = f (θ) curves pass through a marked maximum (θ = 3), whose position depends on the xenon loading.
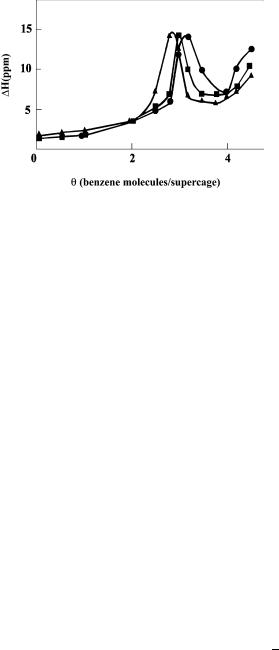
198 |
J.-L. Bonardet et al. |
Fig. 22 The variation of 129Xe linewidth spectra with respect to benzene coverage, θ (average number of benzene molecules per supercage); • PXe = 6.7 kPa; PXe = 40 kPa;PXe = 90 kPa. (Reprinted from [166], with permission from Elsevier Science)
These results are interpreted by the authors as reflecting a mutual hindrance between xenon atoms and benzene molecules, which rearrange by cooperative migration of benzene-Na+ complexes towards the center of the supercages. These results seem to agree with those obtained by Lechert et al. [164] from 1H NMR measurements, by Renouprez et al. [168, 169] from neutron diffraction studies and by de Mallmann and Barthomeuf [165] from IR results. These authors found that benzene molecules start to aggregate at about θ = 2.5. For θ > 4, ∆H increases again with increasing θ, which is consistent with a cooperative interaction of benzene within the limited void space of the supercage. For θ > 4.5, benzene molecules are packed in the supercage into which xenon can no longer enter.
2.4.3
Study of the H2O/Na–Y System
Gédéon et al. [170] have shown that it is possible, in a Na – Y zeolite, to differentiate between water adsorbed in regions accessible or not to xenon, to measure the volume of water in pores and to determine the blockage of windows by water molecules.
Figure 23 shows the variations of δS (δ value for N = 0) and the slope dδ/ dN with the water concentration, C. The decrease in δS with C proves that right from the beginning of dehydration, the number of water molecules per supercage decreases. Furthermore, δS stops decreasing and remains constant when C < 0.15.
This result shows that for 0 < C < 0.15 the mean free path ( ) of xenon at zero concentration is constant. It may be deduced that for C < 0.15 the supercages and the windows between supercages are completely dehydrated. In other words, the water molecules are all in the sodalite cages and the prisms.

NMR of Physisorbed 120XE Used as a Probe to Investigate Molecular Sieves |
199 |
Fig. 23 Effect of the water concentration C [upper (w/w of anhydrous solid); lower (relative concentration)] on shift δN →0 (•) and the slope of the δ = f (N) variations ( ). (Reprinted from [170], with permission from Elsevier Science)
This result is compatible with the fact that each sodalite cage can contain a maximum of four water molecules corresponding to C = 0.15. As for the slope, it remains constant for C < 0.4, whereas δS continues to decrease down to C = 0.15. This means that the void space available to xenon atoms stays about the same ( dδ/ dN constant) but that the mean free path increases (δS decreases) because xenon atoms diffuse more easily between the supercages. It is easy to understand from these results that for 0.15 < C < 0.4 the wa-
ter molecules are in the vicinity of the 8 ˚ apertures, which they obstruct
A
more or less depending on the value of C. Dehydration at these windows does not change the pore volume of the zeolites very much but leads to an easier diffusion of xenon between supercages and therefore greatly affects and δS.
2.4.4
Study of Coke Deposition in Zeolites
The deactivation of zeolites by carbonaceous deposits (coke) is a crucial prob-
lem in industrial cracking, and many studies have shown that deactivation depends on the nature and the structure of the zeolites. 129 Xe NMR spec-
troscopy has proved to be an efficient tool for locating coke inside the cage or
200 |
J.-L. Bonardet et al. |
on the external surface of the zeolite. This technique is also able to show the role of extra-framework aluminum in the cracking activity of zeolites.
The study by Ito et al. [171] concerns coke deposition formed by n-hexane or propylene cracking on HY zeolites. The single 129 Xe NMR line is narrow and almost symmetrical for samples coked with n-hexane; it becomes much broader and asymmetrical for samples coked with propylene, indicating that xenon atoms are much less mobile in the cages. For a sample highly loaded with coke (33% w/w) formed from propylene cracking, the spectrum consists of a broad asymmetrical highly shifted component (157 ppm) and a narrow, almost symmetrical line at ca. 10 ppm. The first line is characteristic of strongly adsorbed xenon atoms in the modified supercages, while the other resonance line may be attributed to xenon atoms adsorbed in mesopores or macrocavities formed by the coke between the crystallites. The δ = f (N) variations show nearly identical slopes for noncoked and 5% coked samples, whereas slopes are much larger for samples coked by propylene to 15 and 33% w/w. These results suggest that the available free volume is slightly reduced when the coke level is low but is seriously affected at high coke loading. By comparing the remaining free volume obtained from the slope and that calculated from the average density of the coke (with the assumption that all the coke is deposited in supercages), the authors show that part of the coke blocks the access to other supercages; in this case, the coke formed must be essentially aromatic or polyaromatic.
Barrage et al. [172, 173] have studied in detail the coke distribution and the influence of dealumination in dealuminated HY and HZSM-5 zeolites. The variations of δ versus [Xe] for dealuminated HY zeolites, which have undergone various hydrothermal treatments, are similar to that described by Ito and Fraissard [98] for Ca – Y or Mg – Y zeolites and by Gédéon et al. [129] for NiY. In all cases, there are adsorption centers stronger than H+ or Na+. The curvature, observed at low xenon concentration, is therefore attributed to the electric field created by nonframework aluminum species equivalent to more or less complexed Al3+ cations (see Sect. 2.3.8). In the case of coked samples, even at low loading, this curvature vanishes (Fig. 24) and δ varies linearly over the entire concentration range. This linearity shows that the coke is deposited firstly on the nonframework aluminum preventing the Al – Xe interactions.
Examination of the δ = f (N) curves for 3 and 10.5% coked samples shows that the slopes increase markedly (by a factor of 2.4) and δN→0 somewhat less. These results mean that, after covering the strong adsorption sites (i.e. extraframework Al), coke forms more homogeneously, affecting both the windows between the α-cages (δS increases) and the volume of the supercages (dδ/ dN increases). When the coke content reaches 15%, the slope is almost identical to that of the 10.5% coked sample but the strong increase of δS shows that the mean free path of xenon is very restricted. Moreover, a second Xe NMR signal appears (45 ppm) independent of the pressure; this is attributed to xenon

NMR of Physisorbed 120XE Used as a Probe to Investigate Molecular Sieves |
201 |
Fig. 24 δ = f (N) variation at 300 K for: HY-reference sample, • HY 3%-coked sample, HY 10.5%-coked sample, HY 15%-coked sample. (Reprinted from [172], with permission from Baltzer Science)
adsorbed in microor meso-cavities of coke formed at the external surface of the crystallites.
A variable-temperature NMR study of adsorption shows that, for the 3% coked sample, δS and dδ/ dN are temperature-dependent (Fig. 25). For each temperature the slope of the δ = f (N) plot is close to that of the straight section of the plot for the reference sample, while the value of δS is higher. The free volume is, therefore, hardly affected but xenon diffusion is restricted. The authors conclude that the coke is located initially at the windows between the supercages. At higher coke content (10.5%), δS and dδ/ dN become independent of the adsorption temperature. This result is similar to that obtained for zeolites with narrow channels. It must be assumed that the high coke content considerably reduces the diameters of the cages and windows, and consequently the mean free path of xenon. The size of the cavities still accessible to the xenon becomes of the same order of magnitude as that of the xenon atoms.
Similar studies have been performed by Tsiao et al. [174] and Liu et al. [175]. The former studied HZSM-5 coking after exposure to 2-butene at 823 K. They found a linear relationship between the chemical shift and the xenon uptake whatever the coke loading. The values of δS are the same for three different coked samples but the slope increases with the amount of coke deposited. They concluded that deactivation results in channel blockage by coke deposition at the openings or at channel intersections. The latter authors investigated the formation of coke in the disproportionation of n-propylbenzene on various zeolites. For HY zeolites their results agree with ours [172]. In the case of HZSM-5 zeolites, they found δ variations analogous

202 |
J.-L. Bonardet et al. |
Fig. 25 δ = f (N) variation on dealuminated HY zeolites. A (3%-coked samples), B (10.5% coked) at different adsorption temperatures: (τ ) 273 K, (•) 300 K, ( ) 319 K, (σ ) 338 K. (Adapted from [172], with permission from Baltzer Science)
to those observed previously by Bonardet et al. [176] after coking by acetone conversion. At low coke loading, they observed two signals which they attributed to two adsorption sites: one in the opening of the pores or midchannel positions, the other at the channel intersections. In our case we also observed, at low coke content, two xenon NMR lines for adsorbed xenon, but we attributed the second signal (which disappears when the coke level increases) to extra-framework aluminum adsorption sites, in agreement with the 27Al NMR spectrum, which shows the presence of octahedrally coordinated aluminum.
It has been also possible to study the reoxidation of coked zeolites. For example, Bonardet et al. [176, 177] have shown that total reoxidation of the carbonaceous residues, even under mild conditions induces structure defects (additional dealumination) and/or partial amorphization leading to a significant loss of internal microporous volume of the catalyst. In the case of highly dealuminated HZSM-5 zeolites (Si/Al > 80) [173] the values of δS obtained are similar for fresh and samples coked during conversion of methanol. Thus, at low concentrations xenon is mainly adsorbed in zones which are not influenced by coke deposits, i.e. which do not contain coke. This result shows that the coke distribution is heterogeneous. A greater slope is observed for the coked samples than for the fresh catalyst, but this slope is the same for both coked samples (7 and 14% w/w). This proves that the available free volume is seriously reduced either by blocking of channels or by deposits in the channel
NMR of Physisorbed 120XE Used as a Probe to Investigate Molecular Sieves |
203 |
intersections. On the other hand, the identical curves for samples with 7 and 14% of coke show that a high proportion of the coke must be located outside the crystallites (at least the part of coke above 7%).
Miller et al. [178] studied the coking of HY zeolites by propylene cracking, but they combined 129 Xe NMR with argon sorption measurements. They found that the increase in xenon chemical shift (in comparison with a fresh sample) depends on the aromaticity of the coke. Moreover, argon sorption showed a bimodal distribution in the pore apertures: one, free of coke (0.72 nm) and the other greatly restricted by the coke deposits (0.58 nm). 129 Xe NMR by itself cannot detect the two environments, because of the rapid exchange of xenon atoms between the two sites.
Finally, comparison of 129 Xe and 1H pulsed-field gradient NMR spectroscopy [179] made it possible to locate the carbonaceous residues on Na – A zeolites and to distinguish unambiguously deposits in the intracrystalline pore system and on the external surface of crystallites. The small decrease in the intracrystalline diffusion coefficient of methane adsorbed in noncoked and coked A zeolites (10–9 to 0.6 × 10–9 m2 s–1) and the marked effect observed on the intracrystalline mean lifetimes (τintra goes from 3 ms to 100 ms) showed that the carbonaceous compounds are mainly deposited as a layer on the external surface, acting as a barrier to molecular mass transfer between the crystallites.
2.5
Study of Supported Metals and Chemisorption Thereon
2.5.1 Introduction
In what follows we shall reason on the basis of faujasite structure zeolites, but the results obtained are easily generalized to other types of solids.
Let us assume now that in various Na – Y zeolite supercages there are distributed solid particles with chemically different surfaces Si. The index, i, denotes a particle type of population ni, among the p types that exist (0 < i < p). Corresponding to each of these populations, there is a shift δi characteristic of the Xe – Si collisions, being the product of two terms:
|
|
Term for the |
|
Term for the |
|
|
δi = |
chemical nature frequency of Xe–Si |
(23) |
||||
|
|
of Si |
|
collisions |
|
|
The spectrum depends on the lifetime of Xe on each adsorption site:
1.If the lifetime of xenon on each surface Si is long (from an NMR point of view), the spectrum of the adsorbed xenon should theoretically contain as many components δi as there are target types, the intensity of each

204 |
J.-L. Bonardet et al. |
being proportional to the number, ni, of targets i, of the same type, the total being p + 1, since the component (shift δNa–Y) due to xenon striking the supercage surfaces and the other Xe must also be taken into account. This situation could be obtained by recording the spectrum at sufficiently low temperature, but the components would be very broad and almost undetectable by classical NMR methods.
2.In the opposite case, where the xenon has a very short lifetime at each adsorption site and can, moreover, diffuse rapidly across several supercages contained in the same crystallite of the zeolite, all the above signals coalesce. The spectrum consists of only one component whose position depends on the values of δi, each weighted by the probability αi of Xe – Si collision:
δ = αiδi + αNa–YδNa–Y with |
αi + αNa–Y = 1. |
(24) |
In this case, it is, of course, very difficult to get much information about this system from this single line. Fortunately, the spectrum depends also on the distribution of the different particles i, j... inside the zeolite crystallites.
Among the different models considered [180, 181] we should note the following case, which is fundamental in the study of chemisorption on metalloaded zeolites (Fig. 26). Let us suppose that in each zeolite crystallite the particles i, j... (with surfaces Si, Sj...) are grouped according to their nature in volumes Vi, Vj... Unless the concentration of one of the species is really very small, the quantity of xenon exchanging between two adjacent zones Vi and Vj is negligible compared to the quantities of xenon in each zone. The system appears then as though there were several independent samples. The NMR spectrum must therefore contain p components corresponding to each type of target, with shift δi, such that:
δi = λiδi + µiδNa–Y. |
(25) |
Fig. 26 Model of distribution of Si and Sj particles grouped according to their nature in volumes Vi and Vj